In principle, the function of an audio power amplifier is a simple one:
to convert a low-power input voltage signal from a pre-amplifier or other
signal source into a higher power signal capable of driving a loudspeaker
or other output load, and to perform this amplification with the least possible
alteration of the input waveform or to the gain/frequency or phase/frequency
relationships of the input signal.
VALVE (VACUUM TUBE) AMPLIFIER DESIGNS
In the earlier days of audio amplifiers, this function was performed by a
two- or three-valve (tube) circuit design employing a single output triode
or pentode, transformer coupled to the load, and offering typical harmonic
distortion levels, at full power -- which would be, perhaps, in the range
2-8 W -- of the order of 5-10% THD, at 1 kHz, and amplifier output stages
of this kind formed the mainstay of radio receivers and radiograms in the
period preceding and immediately following the Second World War.
With the improvement in the quality of phonograph recordings and radio broadcasts
following the end of the war, and the development of the new 'beam tetrode'
as a replacement for the output pentode valve, the simple 'single-ended' triode
or pentode output stage was replaced by push-pull output stages. These used
beam tetrodes either triode-connected, as in the celebrated Williamson design,
or with a more complex output transformer design, as in the so-called Ultra-linear
layout, or in the Quad design, shown in Figs 1(a), (b) and (c). The beam tetrode
valve construction, largely the work of the Marconi Osram valve company of
London, offered a considerable improvement in the distortion characteristics
of the output pentode, while retaining the greater efficiency of that valve
in comparison with the output triode. This advantage in efficiency was largely
retained when the second grid was connected to the anode so that it operated
as a triode.
This electrode interconnection was adopted in the Williamson design, shown
in FIG. 2, in which a substantial amount of overall negative feedback was
employed, to give a harmonic distortion figure of better than 0.1%, at the
rated 15 W output power.
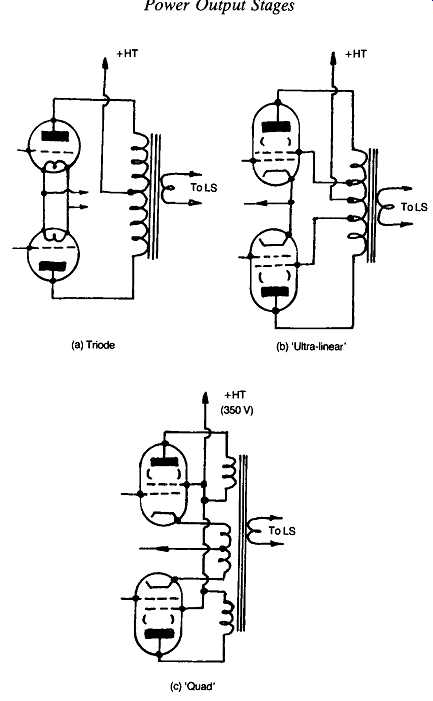
FIG. 1 Push-pull valve amplifier output stages. (a) Triode (b) 'Ultra-linear'
(c) 'Quad'.
In general, the principle quality determining factor of such an audio amplifier
was the output transformer, which coupled the relatively high output impedance
of the output valves to the low impedance of the loudspeaker load, and good
performance demanded a carefully designed and made component for this position.
Nevertheless, such valve amplifier designs did give an excellent audio performance,
and even attract a nostalgic following to this day.
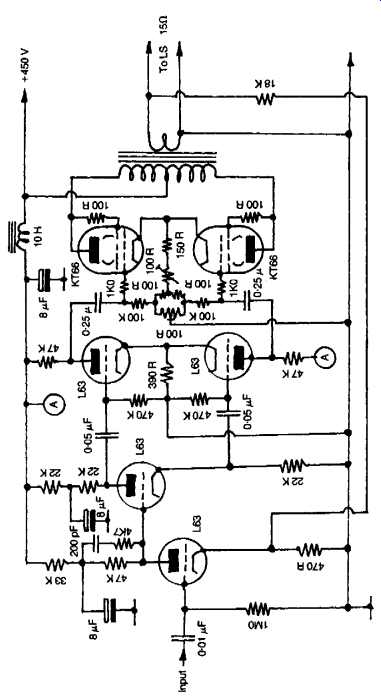
FIG. 2 The Williamson amplifier.
EARLY TRANSISTOR CIRCUITS
With the advent of semiconductors with significant power handling capacity,
and the growing confidence of designers in their use, transistor audio amplifier
designs began to emerge, largely based on the 'quasi complementary' output
transistor configuration designed by HC Lin, which is shown in FIG. 3. This
allowed the construction of a push-pull power output stage in which only PNP
power transistors were employed, these being the only kind then available.
It was intended basically for use with the earlier diffused junction Germanium
transistors, with which it worked adequately well.
However, Germanium power transistors at that time had both a limited power
capability and a questionable reliability, due to their intolerance of junction
temperatures much in excess of 100° C. The availability of silicon power transistors,
which did not suffer from this problem to such a marked degree, prompted the
injudicious appearance of a crop of solid state audio amplifiers from manufacturers
whose valve operated designs had enjoyed an erstwhile high reputation in this
field.
LISTENER FATIGUE AND CROSSOVER DISTORTION
Fairly rapidly after these new semiconductor-based audio amplifiers were
introduced, murmurings of discontent began to be heard from their users, who
claimed, quite justifiably, that these amplifiers did not give the same warmth
and fullness of tone as the previous valve designs, and the term 'listener
fatigue' was coined to describe the user's reaction.
The problem, in this case, was a simple one. In all previous audio amplifier
experience, it had been found to be sufficient to measure the distortion and
other performance characteristics at full output power, since it could reasonably
be assumed that the performance of the amplifier would improve as the power
level was reduced. This was not true for these early transistor audio amplifiers,
in which the performance at full power was probably the best it would ever
give.
This circumstance arose because, although the power transistors were capable
of increased thermal dissipation, it was still inadequate to allow the output
devices to be operated in the 'Class A' mode, always employed in conventional
valve operated power output stages, in which the quiescent operating current,
under zero output power conditions, was the same as that at maximum output.
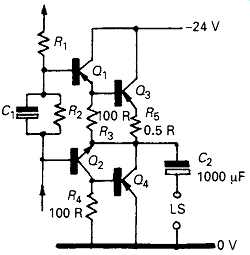
FIG. 3 Quasi-complementary push-pull transistor output stage from HC Lin.
Crossover problems
It was normal practice, therefore, with transistor designs, to bias the output
devices into 'Class B' or ' Class AB', in which the quiescent current was
either zero, or substantially less than that at full output power, in order
to increase the operating efficiency, and lessen the thermal dissipation of
the amplifier.
This led to the type of defect known as 'crossover distortion', which is
an inherent problem in any class 'B' or ‘AB' output stage, and is conspicuous
in silicon junction transistors, because of the abrupt turn-on of output current
with increasing base voltage, of the form shown in FIG. 4.
In a push-pull output stage employing such devices, the transfer characteristics
would be as shown in FIG. 5(b), rather than the ideal straight line transfer
of FIG. 5(a). This problem is worsened anyway, in the quasi-complementary
Lin design, because the slopes of the upper and lower transfer characteristics
are different, as shown in FIG. 5(c). The resulting kink in the transfer characteristic
shown in FIG. 5(d) lessens or removes the gain of the amplifier for low-level
signals which cause only a limited excursion on either side of the zero voltage
axis, and leads to a 'thin' sound from the amplifier.
It also leads to a distortion characteristic which is substantially worse
at low power levels - at which much listening actually takes place - than
at full power output. Moreover, the type of distortion products generated
by crossover distortion comprise the dissonant seventh, ninth, eleventh and
thirteenth harmonics, which are much more objectionable, audibly, than the
second and third harmonics associated with the previous valve amplifier designs.
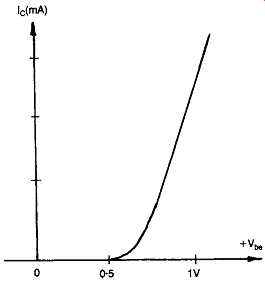
FIG. 4 Turn-on characteristics of silicon junction transistor.
Additionally, the absence of the output transformer from such transistor
amplifier designs allowed the designers to employ much greater amounts of
negative feedback (NFB) from the output to the input of the circuit, to attempt
to remedy the non-linear transfer characteristics of the system.
This increased level of NFB impaired both the load stability and the transient
response of the amplifier, which led to an 'edgy' sound. High levels of NFB
also led to a more precise and rigid overload clipping level.
Such a proneness to 'hard' clipping also impaired the sound quality of the
unit.
This failure on the part of the amplifier manufacturers to test their designs
adequately before offering them for sale in what must be seen in retrospect
as a headlong rush to offer new technology is to be deplored for several reasons.
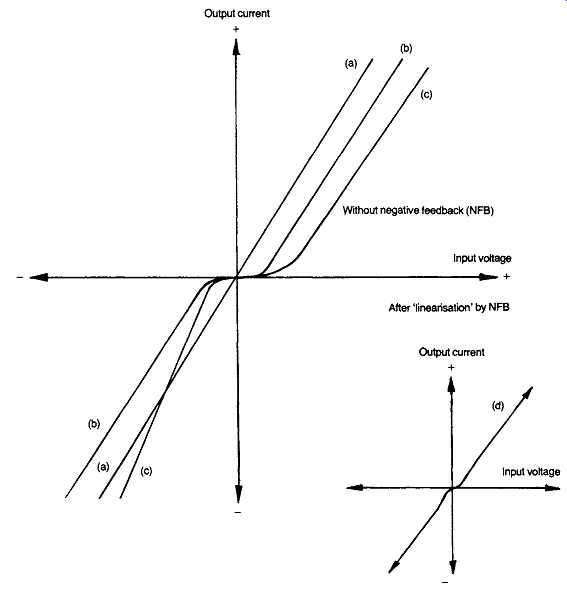
FIG. 5 Transistor ' Class AB' push-pull output stage characteristics. (Line
'a' is a straight line for comparison.)
Of these, the first is that it saddled many unsuspecting members of the public
with equipment which was unsatisfactory, or indeed unpleasant, in use. The
second is that it tended to undermine the confidence of the lay user in the
value of specifications - simply because the right tests were not made, and
the correct parameters were left unspecified. Finally, it allowed a 'foot
in the door' for those whose training was not in the field of engineering,
and who believed that technology was too important to be left to the technologists.
The growth of this latter belief, with its emphasis on subjective assessments
made by self-appointed pundits, has exerted a confusing influence on the whole
field of audio engineering, it has led to irrationally based choices in consumer
purchases and preferences, so that many of the developments in audio engineering
over the past two decades, which have been substantial, have occurred in spite
of, and frequently in the face of, well-publicized and hostile opposition
from these critics.
IMPROVED TRANSISTOR AMPLIFIER DESIGNS
The two major developments which occurred in transistor output circuit technology,
which allowed the design of transistor power amplifiers which had a low inherent
level of crossover distortion, were the introduction in the mid 1960s of fully
complementary (NPN and PNP) output power transistors by Motorola Semiconductors
Inc, and the invention by IM Shaw (Wireless World, June 1969), subsequently
refined by P. J. Baxandall (Wireless World, September 1969) of a simple circuit
modification to the basic quasi-complementary output push-pull pair to increase
its symmetry.
These layouts are shown in Figs 6(a) and (b). The modified version shown
in FIG. 6(c) is that used by the author in his Hi-Fi News 75 W power amplifier
design , for which the complete circuit is given in FIG.
In many ways the Shaw/Baxandall quasi-complementary output transistor circuit
is preferable to the use of fully complementary output transistors, since,
to quote J. Vereker of Naim Audio, NPN and PNP power transistors are only
really as equivalent as a man and a woman of the same weight and height' -
the problem being that the different distribution of the N- and P doped layers
leads to significant differences in the HF performance of the devices. Thus,
although the circuit may have a good symmetry at low frequencies, it becomes
progressively less symmetrical with increasing operating frequency.
With modern transistor types, having a higher current gain transition frequency
(that frequency at which the current gain decreases to unity), the HF symmetry
of fully complementary output stages is improved, but it’s still less good
than desired, so the relative frequency/phase characteristics of each half
of the driver circuit may need to be adjusted to obtain optimum performance.
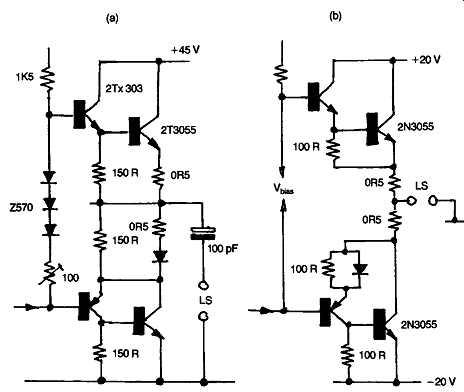
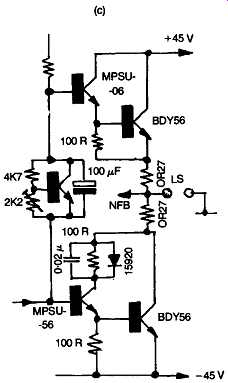
FIG. 6 Improved push-pull transistor output stages.
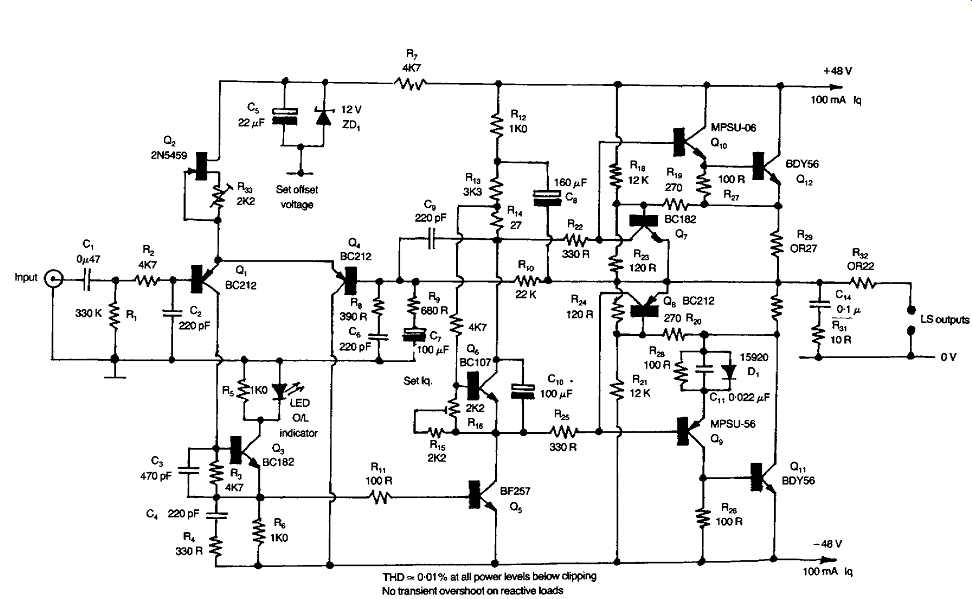
FIG. 7 JLH Hi-Fi News 75 W audio amplifier.
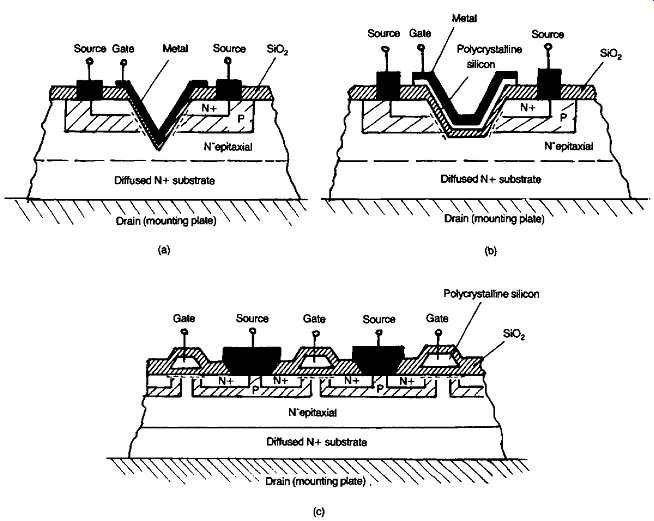
FIG. 8 Power MOSFET structure, (a) V-MOSFET, (b) U-MOSFET, (c) D-MOSFET.
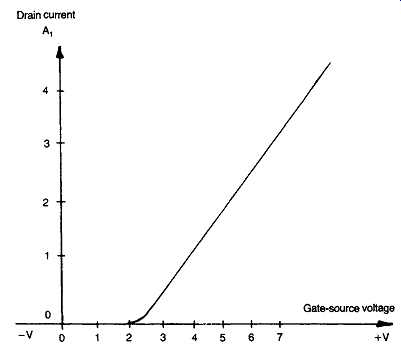
FIG. 9 Power MOSFET operating characteristics.
POWER MOSFETs
These transistor types, whose operation is based on the mobility of electrostatically
induced charge layers (electrons or 'holes') through a very narrow layer of
undoped or carrier-depleted silicon, have a greatly superior performance to
that of bipolar junction transistors, both in respect of maximum operating
frequency and linearity, and allow considerable improvements in power amplifier
performance for any given degree of circuit complexity.
Two forms of construction are currently employed for power MOSFETs, the vertical
or 'V MOSFET, which can employ either a 'V’ or a 'U' groove formation, of
the types shown in Figs 8(a) and (b); though, of these two, the latter is
preferred because of the lower electrostatic stress levels associated with
its flat-bottomed groove formation; or the 'D' MOSFET shown in FIG. 8(c).
These devices are typically of the 'enhancement' type, in which no current
flows at zero gate/source voltage, but which begin to conduct, progressively,
as the forward gate voltage is increased. Once the conduction region has been
reached, the relationship between gate voltage and drain current is very linear,
as shown.
By comparison with 'bipolar' junction power transistors, of conventional
types, the MOSFET, which can be made in both N channel and P channel types
to allow symmetrical circuit layout - though there is a more limited choice
of P channel devices - does not suffer from stored charge effects which limit
the 'turn-off speed of bipolar transistors.
The greater speed and lesser internal phase shifts of power amplifiers based
on power MOSFETs allow greater freedom in the design of overall NFB layouts.
This in turn, gives superior performance in the middle to upper frequency
range. Greater care is needed, in the circuit design, however to avoid high
frequency parasitic oscillation, which can cause rapid failure of the devices.
The principal technical problem in the evolution of power transistors derived
from these charge operated devices, as distinct from the existing small signal
MOSFETs, lies in obtaining an adequate capacity for current flow through the
device. This problem is solved in two ways; by making the conduction path
through which the current must flow as short as possible, and by fabricating
a multiplicity of conducting elements on the surface of the silicon slice,
which can be connected in parallel to lower the conducting resistance of the
device.
V and U types
In the 'V’ or 'U' groove MOSFET, taking the case of the '?' channel devices
shown in FIG. 8, the required narrow conduction channel, in which electrostatically
formed negative charges (electrons) may be induced in the depleted T' type
layer, is obtained by etching a channel through a previously diffused, or
epitaxially grown, pair of differently doped layers.
With modern technology the effective thickness of such layers can be controlled
with great precision.
In the case of the D-MOS device of FIG. 8(c), the required narrow channel
length is achieved by the very precise geometry of the diffusion masks used
to position the doped regions. It’s customary in the D-MOS devices to use
a polycrystalline silicon conducting layer, rather than aluminum, to provide
the gate electrode, since this offers a lower likelihood of contamination
of the thin gate insulating layer.
In all of these MOS devices, the method of operation is that a voltage applied
to the gate electrode will cause a charge to be induced in the semiconductor
layer immediately below it, which, since this layer of charge is mobile, will
cause the device to conduct.
Both for the 'V/U' MOS and the D' MOS devices, the voltage breakdown threshold
of the gate insulating layer, which must be thin if the device is to operate
at all, will be in the range 15-35 V. It can be seen that part of this gate
insulating layer lies between the gate electrode and the relatively high voltage
'drain' electrode. Avoidance of gate/drain voltage breakdown depends therefore
on there being an adequate voltage drop across the N-doped intervening drain
region. This in turn depends on the total current flow through the device.
This has the effect that the actual gate/drain breakdown voltage is output
current-dependent, and that some protective circuitry may need to be used,
as in the case of bipolar output transistors, if damage is to be avoided.
OUTPUT TRANSISTOR PROTECTION
An inconvenient characteristic of all junction transistors is that the forward
voltage of the P-N junction decreases as its temperature is increased. This
leads to the problem that if the current through the device is high enough
to cause significant heating of the base-emitter region, the forward voltage
drop will decrease. If, due to fortuitous variations in the thickness of this
layer or in its doping level, some regions of this junction area heat up more
than others, then the forward voltage drop of these areas will be less, and
current will tend to be funneled through these areas causing yet further heating.
This causes the problem known as 'thermal runaway' and the phenomenon of
secondary breakdown, if certain products of operating current and voltage
are exceeded. The permitted regions of operation for any particular bipolar
transistor type will be specified by the manufacturers in a 'safe operating
area' (SOA) curve, of the type shown in FIG. 10.
The circuit designer must take care that these safe operating conditions
are not exceeded in use , for example by the inadvertent operation of the
amplifier into a short-circuited output load. Provided that the SO A limits
are not greatly exceeded, a simple fuse on the output circuit will probably
be adequate, but a more effective type of protection is that given by the
clamp transistors, Q7 and Q8 in the power amplifier design shown in FIG.
In this circuit arrangement, designed by A. R. Bailey, the clamp transistors
monitor simultaneously the voltage present across the output transistors,
by means , for example, of R18 and R23, and also the output current, in the
case of the upper output transistor, by monitoring the voltage developed across
R2ç. If the combination of these two voltage contributions exceeds the 0.55
V turn-on voltage of the clamp transistor, (Q7), it will conduct and shunt
the input signal applied to the first transistor of the output pair (Q10)
and avoid output transistor damage.
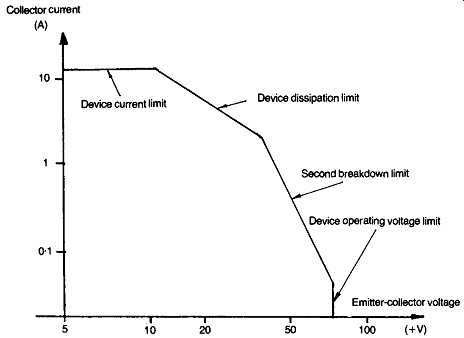
FIG. 10 Typical safe operating area (SOA) curve for junction power transistor.
In the case of power MOSFETs, a simple zener diode, connected to limit the
maximum forward voltage which can be applied to the output device, may be
quite adequate. An example of this type of output stage protection is shown
in the circuit for the output stages of a 45 W power MOSFET amplifier designed
by the author, shown in FIG. 11.
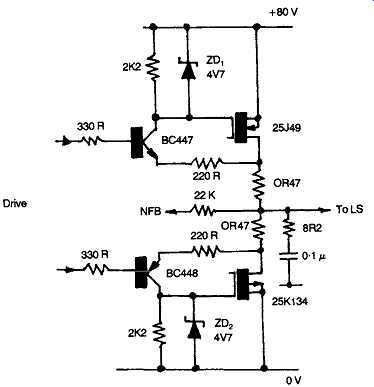
FIG. 11 Zener diode protection for power MOSFET output stage.
POWER OUTPUT AND POWER DISSIPATION
One of the most significant changes in audio technology during the past 30
years has been in the design of loudspeaker units, in which the electro acoustic
efficiency, in terms of the output sound level for a given electrical power
input, has been progressively traded off against flatness of frequency response
and reduced coloration.
This is particularly notable in respect of the low-frequency extension of
the LS response, in closed box 'infinite baffle' systems. Among other changes
here, the use of more massive bass driver diaphragms to lower the fundamental
resonant frequency of the system - below which a - 12 dB/octave fall-off in
sound output will occur - requires that more input power is required to produce
the same diaphragm acceleration.
On the credit side, the power handling capacity of modern LS systems has
also been increased, so that equivalent or greater sound output levels are
still obtainable, but at the cost of much greater input power levels.
Power levels
As a specific example of the power levels which may be needed for realistic
reproduction of live sounds using typical modern LS units, recent measurements
made in a recording studio showed that the peak output power required from
the amplifier to match the peak sound levels produced by a grand piano played
by a professional musician in the same room, was in excess of 300 W per channel.
This test was made using a pair of high quality monitor speakers, of a kind
also widely used in domestic hi-fi setups, whose overall efficiency was typical
of modern designs.
No conventional valve amplifier, operating in 'Class A', could meet this
power output requirement, in a stereo system, without the penalties incurred
in terms of size, cost, and heat output being intolerable. In contrast the
solid-state class AB power amplifier actually used in this test fitted comfortably
into a 7 in. high slot in a standard 19 in. rack, and ran cool in use.
This reduction in LS efficiency has led to compensatory increases in power
amplifier capability, so that the typical output power of a contemporary domestic
audio amplifier will be in the range 50-100 W, measured usually with a 4 or
8 ohm resistive load, with 25 or 30 W units, which would at one time have
been thought to be very high power units, now being restricted to budget priced
'mini' systems.
Design requirements
The design requirements for such power output levels may be seen by considering
the case of a 100 W power amplifier using junction transistors in an output
stage circuit of the form shown in FIG. 12, the current demand is given by
the formula.
/ = ?/PÎR
where P = 100, and R = 8.
This gives an output current value of 3.53 A (RMS), equivalent to a peak
current of 5 A for each half cycle of a sinusoidal output signal. At this
current the required base-emitter potential for Q3 will be about 3 V, and
allowing for the collector-emitter voltage drop of Q1? the expected emitter-collector
voltage in Q3 will be of the order of 5V.
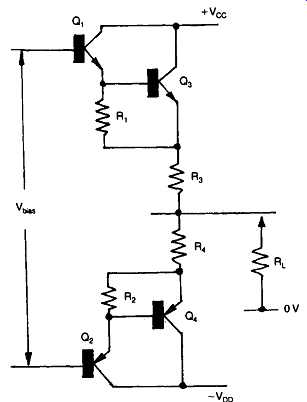
FIG. 12 Typical transistor output stage.
In an 8 ohm load, a peak current of 5 A will lead to a peak voltage, in each
half cycle, of 40 V. Adding the 1.1 V drop across R3, it can be seen that
the minimum supply voltage for the positive line supply must be at least 46.1
V. Since the circuit is symmetrical, the same calculations will apply to the
potential of the negative supply line. However, in any practical design, the
supply voltages chosen will be somewhat greater than the calculated minimum,
so line voltages of ±50 V would probably be used.
For a true 'Class A' system, in which both of the output transistors remained
in conduction for the whole output cycle, an operating current of at least
5 A, DC, would be required, leading to an output stage dissipation , for each
channel, of 500 W. In the case of a typical ' Class AB' output stage, with
a quiescent current of 100 mA, a much more modest no-signal dissipation of
10 W would be required. At full power, the device dissipation may be calculated
from the consideration that the RMS current into the load, in each half cycle,
will be 3.53 A giving an RMS voltage swing of 28.24 V. The mean voltage across
each device during the half cycle of its conduction would therefore be 50
minus 28.24, and the mean dissipation , for each half of the power output
stage would be 76.8 W. The worst case dissipation for such output stages,
depending on supply line voltage margins, will be at about half power, where
the dissipation in each half of the output stage could rise to 80-85 W, and
the heat sinking arrangements provided must be adequate to meet this need.
It’s still, however, only a third of that required by a 'Class A' system,
and even then only required on power peaks, which, with typical program material,
occur infrequently so that the average output stage heat dissipation, even
under conditions where the amplifier is used at near its rated maximum power
output, may well only be 20-30 W.
GENERAL DESIGN CONSIDERATIONS
Some of the techniques used for obtaining high gain and good linearity from
a voltage amplifier stage, using semiconductors, were discussed in SECTION
4. However, in the case of low-power voltage amplifier stages, all of the
gain elements will be operated in 'Class A', so that there won’t be a need
to employ large amounts of overall negative feedback (NFB) to assist in linearizing
the operating characteristics of the stage.
In power amplifier circuits, on the other hand, the output devices will almost
certainly be operated in a non-linear part of their characteristics, where
a higher level of NFB will be needed to reduce the associated distortion components
to an acceptable level.
Other approaches have been adopted in the case of power amplifier circuits,
such as the feed-forward of the error signal, or the division of the output
stage into low power 'Class A' and high power 'Class B' sections.
These techniques will be discussed later. Nevertheless, even in these more
sophisticated designs, overall NFB will still be employed, and the problems
inherent in its use must be solved if a satisfactory performance is to be
obtained.
Of these problems, the first and most immediate is that of feedback loop
stability. The Nyquist criterion for stability in any system employing negative
feedback is that, allowing for any attenuation in the feedback path, the loop
gain of the system must be less than unity at any frequency at which the loop
phase shift reaches 180°.
Bode Plot
This requirement is most easily shown in the 'Bode Plot' of gain versus frequency
illustrated in FIG. 13. In the case of a direct-coupled amplifier circuit,
and most modern transistor systems will be of this type, the low frequency
phase lead is unlikely to exceed 90°, even at very low frequencies, but at
the higher frequency end of the spectrum the phase lag of the output voltage
in relation to the input signal will certainly be greater than 180° at the
critical unity gain frequency, in any amplifier employing more than two operational
stages, unless some remedial action is taken.
In the case of the simple power amplifier circuit shown schematically in
FIG. 14, the input long-tailed pair, Ch and Q2, with its associated constant
current source, CQ, and the second stage, 'Class A' amplifier, Q3 with its
constant current load, CC2 , form the two voltage gain stages, with Q4 and
Q5 acting as emitter-followers to transform the circuit output impedance down
to a level which is low enough to drive a loudspeaker (Z1). In any amplifier
having an output power in excess of a few watts, the output devices, Q4 and
Q5, will almost certainly be elaborated to one of the forms shown in Figs
3 or 6, and, without some corrective action, the overall phase shift of the
amplifier will certainly be of the order of 270°, at the critical unity gain
point. This means that if overall negative feedback were to be employed, via
R5 and R4, the circuit would oscillate vigorously at some lower, 180° phase-shift
frequency.
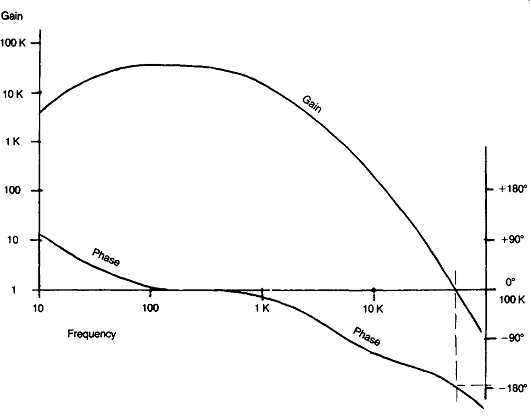
FIG. 13 Bode Plot relating gain and phase to frequency in notional marginally
stable amplifier.
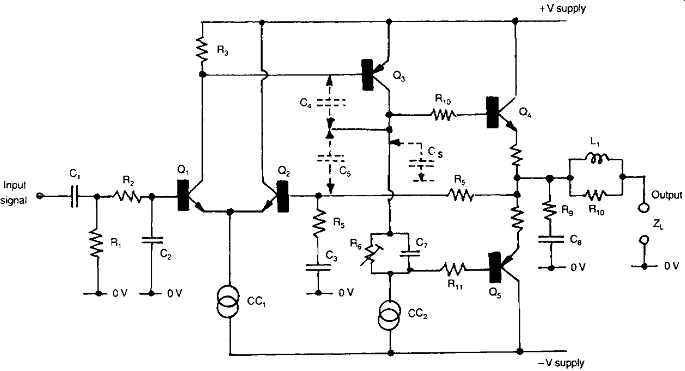
FIG. 14 Basic layout of simple audio amplifier.
SLEW-RATE LIMITING AND THD
Until a few years ago, the most common way of stabilizing a negative feedback
amplifier of this type was by means of a small capacitor, C4, connected across
the second amplifier transistor, Q3. This particularly appealed to the circuit
designers because it allowed the circuit to be stabilized while retaining
a high level of closed-loop gain up to a high operating frequency, and this
facilitated the attainment of low overall harmonic distortion figures at the
upper end of the audio frequency band.
Unfortunately, the use of an HF loop stabilization capacitor in this position
gave rise to the problem of slew-rate limiting, because there is only a finite
rate at which such a capacitor could charge through the constant current source,
CC2, or discharge through the current available at the collector of Q^
Slew limiting
This leads to the type of phenomenon shown in FIG. 15, in which, if a continuous
signal occurs at the same time as one which leads to a sudden step in the
mean voltage level - which could happen readily in certain types of program
material - then the continuous signal will either be mutilated or totally
lost during the period during which the amplifier output voltage traverses,
(slews), under slew-rate limited conditions, between output levels '1' and
'2'.
This type of problem had been well known among amplifier designers for many
years, and the deleterious effect upon the sound of the amplifier had also
been appreciated by those who were concerned about this point.
However, the commercial pressures upon designers to offer circuits which
met the reviewers magic specification of 'less that 0.02% THD from 20 Hz to
20 kHz', led to many of the less careful, or more cynical, to use the slew-rate
limiting system of HF stabilization, regardless of the final sound quality.
Public attention was drawn to this phenomenon by M. Otala, in a paper in
September 1970 (IEE Trans. AU-18, No 3), and the subsequent discussions and
correspondence on this point were valuable in convincing the reviewers that
low levels of harmonic distortion, on their own, were an insufficient guarantee
of audio quality.
HF stabilization
The alternative method of HF stabilization which could be employed in the
circuit of FIG. 14, and which does not lead to the same problems of slew-rate
limitation - in that it causes the HF gain to decrease throughout the amplifier
as a whole, rather than leaving the input stage operating without feedback
at all during the slew-rate limited period - is by the use of capacitor between
Q3 collector, and Q2 base, as indicated by C5, and as used in the author's
15-20 W 'class AB' design (Wireless World, July 1970).
Unfortunately, although this type of stabilization leads to designs with
pleasing sound quality, it does not lead to the same degree of distortion
reduction at the HF end of the spectrum, unless a higher level of loop gain
is employed. The 75 W design shown in FIG. 7, which achieves a typical distortion
performance of less than 0.015% over the whole audio spectrum, is a good example
of this latter approach.
A useful analysis of the problem of slew-rate induced distortion, in non-mathematical
terms, was given by W. G. Jung, in Hi-Fi News (Nov. 1977), with some additional
remedial circuit possibilities shown by the author in the same journal in
January 1978.
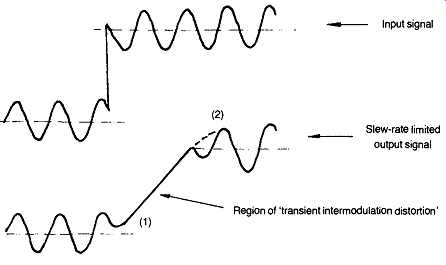
FIG. 15 Effect of slew-rate limiting on amplifier handling combined signals.
Zobel network
Other components used for correcting the phase characteristics of the feedback
loop are the so-called Zobel network (C8, R9) connected across the output,
which will help avoid instability with an open-circuit load, and the inductor/resistor
network (Lj, R10) in series with the load, which is essential in some designs
to avoid instability on a capacitative load.
Since Lj is a very small inductor, typically having a value of 4.7 microhenries,
its acoustic effects within the 20-20 kHz band will usually be inaudible.
Its presence will, however, spoil the shape of any square wave test waveform,
as displayed on an oscilloscope, especially with a capacitative simulated
LS load.
Bearing in mind that all of these slew-rate and transient waveform defects
are worse at higher rates of change of the input signal waveform, it’s prudent
to include an input integrating network, such as R2/C2 in FIG. 14, to limit
the possible rate of change of the input signal, and such an input network
is common on all contemporary designs. Since the ear is seldom sensitive beyond
20 kHz, except in young children, and most adults' hearing fails below this
frequency, there seems little point in trying to exceed this HF bandwidth,
except for the purpose of producing an impressive paper specification.
A further significant cause of slew-rate limitation is that of the stray
capacitances (Cs) associated with the collector circuitry of Q3, in Fig. 14,
and the base input capacitance of Q4/Q5· This stray capacitance can be charged
rapidly through Q3, but can only be discharged again at a rate determined
by the output current provided by the current source CC2. This current must
therefore be as large as thermal considerations will allow.
The possible avoidance of this source of slew-rate limitation by fully symmetrical
amplifier systems, as advocated by Hafler, has attracted many designers.
Among the other defects associated with the power output stage, is that of
'hang up' following clipping, of the form shown graphically in Fig.16. This
has the effect of prolonging, and thereby making more audible, the effects
of clipping. It can usually be avoided by the inclusion of a pair of drive
current limiting resistors in the input circuitry of the output transistors,
as shown by R10 and Rn in FIG. 14.
cont. >>
|