The audio enthusiast, like nearly every other human being, takes most, if
not all, his physiological and psychological processes for granted. Indeed,
most of us are typically unaware of those internal events which enable us to
live our lives and experience our environment. Only now and then tingling fingers,
ringing ears, a grumbling stomach or painful joints remind us indirectly of
the specific sensory systems which gather the sensations and information the
brain requires.
Of our 10 or possibly 11 individual sensory systems (each of which has unique,
specialized receptors that respond to different kinds of stimuli), we tend
to think of vision first. Indeed, since the great majority of research into
sensory mechanisms has concerned itself with vision, we have more information
about the visual sense modality than about any of the others. Nevertheless,
hearing is extremely important in the lives of humans and not merely because
we enjoy music. It has been long observed, for example, that deafness typically
has a more disastrous effect on the psychological development of an individual
than does blindness; not being able to communicate aurally, vocally and by
the uttered word isolates us in a way blindness does not.
An audiophile, of course, has a strong interest in the adequate functioning
of his or her auditory system. Knowledge about it, while of intrinsic interest,
can also enhance many listening experiences, as well as provide a broader basis
for the rational selection and evaluation of various stereo components. Information
about auditory mechanisms also will enable one to more adequately care for
this vital and largely irreplacable link in the high-fidelity chain. We will
first consider the auditory system from an anatomical basis, then we will discuss
several physiological mechanisms involved in the processes of hearing. To more
fully appreciate the anatomy and manner of functioning of our hearing system,
let's first briefly discuss the biological origins and embryological development
of the auditory system.
Origin and Development
In the evolutionary or phylogenetic sense, the auditory system is a derivative
of a receptor system originally concerned with balance and equilibrium, not
hearing. This sense modality, which we now call the vestibular sense, responds
to rotation, our orientation in space, balance, acceleration, deceleration,
and similar forces acting on the body. Wild amusement park rides strongly stimulate
the receptors for this sense, which are located in the three-semi-circular
canals shown in Figs. 1 and 2. Vertigo and car-, air- and boat-sickness are
all products of vestibular system functioning. Among animals with backbones,
the vestibular systems of fishes, amphibia (frogs, salamanders), reptiles (snakes,
turtles), birds and mammals are remarkably similar and differ mostly in details
and the degree of refinement. The similarity between the fishes' vestibular
apparatus and our own is apparent in Figs. 1 and 2.
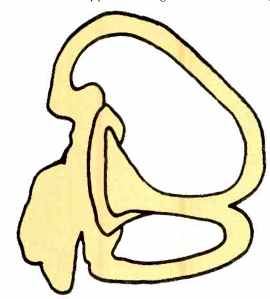
Fig. 1 --Vestibular apparatus of a fish.
The vestibular system of the fish derives from the same group of cells that
form the fishes' lateral line "organ."
This lateral line system of receptors is readily seen on tropical fish and
on many edible varieties at the grocers as a thin line midway down the side
of the fish, which extends from just behind the gillplate to the base of the
fishes' tail. The receptors of this lateral line system of fish are tiny hair
cells, called neuromasts. These hair cells are themselves very similar to and
appear to function much like the hair cells of our inner ear which are the
actual receptors for hearing. References to Fig. 4 will give some idea of these
hair cells in the human inner ear. Thus, the ultimate origin of our hearing
receptors appears to have been a sensory system in the fish, the lateral line
system, with its many hair cells situated in a lengthwise line down the fishes'
side. Our auditory system appears to be a modification of the vestibular apparatus
to accommodate the hair cells of the lateral line system.
Not surprisingly, in view of their relationship, the vestibular and auditory
apparatus in the human develop nearly on a par with one another during gestation.
Of the 12 cranial nerves which directly enter the lower part of the brain,
the eighth cranial nerve is concerned with both hearing and equilibrium. We
call this eighth cranial nerve the statoacoustic nerve to acknowledge its important
equilibrium, vestibular and auditory functions. This nerve carries neural impulses
from the vestibular receptors of the inner ear to the appropriate regions in
the brain many months before the infant is born. In fact, study of human fetuses
indicates that the vestibular receptors respond to stimuli and the statoacoustic
nerve transmits neural impulses generated by these receptors to the brain by
the 12th week of gestation.
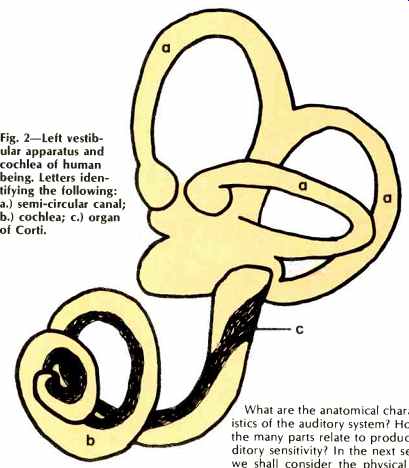 Fig. 2--Left vestibular apparatus and cochlea of human being. Letters identifying
the following: a.) semi-circular canal; b.) cochlea; c.) organ of Corti.
The auditory portions of this system develop more slowly. After 24 to 25 weeks
of growth, the human fetus appears to have an auditory system that is complete
in most details. If not complete, it is, nevertheless, functional at this point
in time. Analyses have shown, for example, that the varied auditory and vestibular
stimuli a fetus receives encourage the growth of the neural centers which receive
these impulses from the statoacoustic nerve. A fetus will typically experience
extensive vestibular stimulation, depending on the mobility of its mother.
The range of auditory experience is restricted, but not eliminated by the niotic
fluid which encompasses the fetus and fills its external auditory meatuses
(air passages to the ear drum). The vestibular and auditory stimuli that impinge
on the fetus and to which it can respond before birth contribute greatly to
the development of both its vestibular and auditory sensory systems. At birth,
then, these sensory systems are totally or highly matured. The receptors and
neural transmission "lines" are complete and sufficiently well-developed
to begin functioning in the outside world at a comparatively sophisticated
level. Numerous studies have shown, for example, that newborn infants, less
than two hours old, respond to a variety of sounds. More recently it has been
shown that an infant is capable of discriminating familiar from unfamiliar
voices within days after birth.
What are the anatomical characteristics of the auditory system? How do the
many parts relate to produce auditory sensitivity? In the next section we shall
consider the physical attributes of our hearing mechanism and the manner in
which the vibrations in air eventually become the auditory experiences that
so enrich our lives.
General Anatomy
Invariably when we think of the ear and of hearing, what first comes to mind
is the accessory structure attached to each side of our heads, the cartilaginous
pinna or auricle which aids in selecting and focusing vibrations at our ear
drum. Some may think also of the tiny bones of the middle ear which transmit
the vibrations of the eardrum to more internally situated structures. However,
even these bones, called ossicles, are accessories-items convenient for but
not essential to hearing in vertebrates. In a sense, the pinna and ossicles
perform a preamp function-equalization and first-stage amplification. The real "ear" is
the internal or inner ear, a coiled structure situated in an appropriately
coiled canal within the temporal bone of the head. This coiled structure, called
the cochlea, is shown in Fig. 2. In humans, the cochlea and its canal are coiled
2 3/4 times. The auditory sense receptors, the microscopic hair cells of Fig.
4, are situated here within part of the cochlea from one end of the spiral
to the other.
Thus, the pinna, auditory canal, eardrum, and ossicles enhance our ability
to hear, but they are not the auditory receptors. To be sure, rupture of the
eardrum and disruption of the three ossicles would result in a catastrophe
from the audio enthusiast's point of view-a hearing deficit of from 30- to
50 decibels magnitude, but not complete deafness.
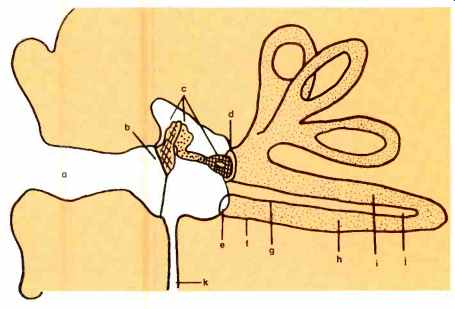 Fig. 3--Diagram of mammalian auditory apparatus. Letters identify the following:
a.) auditory meatus; b.) eardrum; c.) ossicles; d.) oval window; e.) round
window; f.) uncoiled cochlea; g.) basilar membrane; h.) tympanic chamber; i.)
vestibular chamber; j.) middle chamber; k.) eustachian tube.
Outer and Middle Ear
The relatively simple anatomy of the outer and middle ear doesn't show the
complexity of problems their evolutionary development has solved. Reference
to Fig. 3 will make clear the fact that the pinna and external auditory meatus
are a funnel.
The human meatus is about one inch long with a natural vibration frequency
of just less than 4000 Hz. The large amplitude sound pressure waves in air
are funneled to the eardrum or tympanic membrane. The eardrum, remarkably,
manages to reflect back very little incident energy and to transmit most of
it. Frequencies up to 2000 Hz cause the eardrum to vibrate as a whole, like
a disc, with maximum oscillation at the bottom of the drum.
As the frequency increases, the mode of vibration becomes increasingly complex
with the drumhead no longer vibrating as a whole. The three ossicles, attached
to flexible membranes at both input and output, transform the high amplitude,
low pressure input movements of the eardrum into low amplitude, high pressure
movements at the output point of the ossicles, the footplate of the stirrup
(see Fig. 3); they function as a transformer.
Pressure at the eardrum (where it begins) is approximately five percent of
the output pressure transmitted from the stirrup footplate to the oval window
of the cochlea, the other flexible membrane (Fig. 3). As you might imagine,
such a mechanical advantage could cause damage. To avert this, the two smallest
striated muscles in the entire body are attached to two ossicles, respectively.
These two muscles protect the hair cells from most overly loud noises and the
huge osscilations derived from them by increasing the internal friction of
the system, thus reducing the ease of sound transmission. However, some intense
noises (usually man-made) may have such an abrupt wavefront as to reach the
hair cells unattenuated because these small muscles require between 60-150
milliseconds to contract. Permanent damage to hair cells is the result in this
case; they are simply sheared off. The behavioral effect is a frequency region
where one does not hear any sounds at all. Fortunately, most sounds are frequency
composites or many of us would have more difficulty than we do.
The fantastic sensitivity of the ear can be attributed to nature's use of
several transformers--each of which largely ameliorates the energy losses at
the several "interfaces" in the auditory receptive system. The first
transformer between the air and the cochlear fluid is an hydraulic piston transformer.
It includes the eardrum, the ossicles, the oval window, and the inner ear fluid.
Calculations by Dr. Georg von Bekesy (1961 Nobel Prize winner for his audition
research) indicate that the middle ear anatomy does, in fact, minimize energy
loss in passing vibrations to the cochlea. Measurement in a variety of mammals
has shown, also, that absolute auditory thresholds (minimal required pressure
sensitivity to produce a neuro-electric response) are nearly equal.
That is, little more energy can be conserved for transmission by further modifications
to this already highly efficient system. This highly evolved capacity to transmit
rather than to reflect incident energy is the special attribute of the outer
and middle ear.
The very small inertia of the ossicles provides one basis for this capacity.
Of course, high signal sensitivity implies high sensitivity to noise as well.
Fortunately, the ear does establish and maintain an adequate signal-to-noise
ratio. The effects of the many internal sources of noise (e.g. vocalization,
chewing, breathing, muscular contraction, circulation of blood, and neural
discharge) appear to have been minimized by judicious placement of sensitive
components. External sources of noises must, of course, be handled differently.
It is known presently, for example, that the S/N ratio is improved at several
levels of the auditory nervous chain. A primary mechanism involved here is
the inhibition of that nervous discharge which is largely random (as opposed
to periodic) as is the frequency of most noise, a common mode rejection of
sorts.
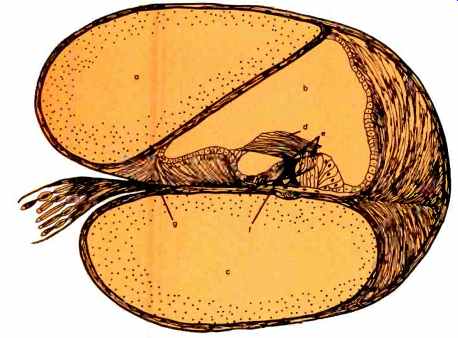
Fig. 4--Cross section through human cochlea. Letters identify: a.) vestibular
chamber; b.) middle chamber; c.) tympanic membrane; g.) nerve fibers.
Once the energy has reached the inner ear, a second transformer comes into
play. This transformer utilizes vertical pressure on a flexible membrane, the
basilar membrane, to create a longitudinal stress in a second, inflexible membrane,
the tectorial membrane (Fig. 4). The actual receptors, the hair cells, being
situated somewhat obliquely between these two membranes, as shown, have their
hairs bent over at the point where they contact the stiff, tectorial membrane
by the shearing force thus generated. This second transformer has been called
a shearing transformer.
Let us look more closely at the elements of this mechanism and their interrelations.
About 12,000 hair cells (that's all) are situated within the spiral cochlea
(Figs. 2 and 3). The cochlea is divided by two membranes into three chambers,
two of which communicate with one another at the tip of the cochlea, through
the helicotrema. These two chambers, the "upper" or scala tympani
and the "lower" or scala vestibule, are filled with a fluid, perilymph
(Fig. 3), which is nearly identical to the watery fluid that baths the central
nervous system, called cerebrospinal fluid. The third, non-communicating chamber,
often called the cochlear duct, is between the other two chambers. Fluid fills
this chamber also. This fluid, termed endolymph, is more viscous than the perilymph
of the two other connecting chambers. The ossicular vibrations are transmitted
to the fluid of the upper- and lower-connecting chambers by the stirrup which
rests against another membrane, the oval window. Since these chambers are completely
filled with watery perilymph fluid, the fluid pushes out on all the boundary
surfaces. One of these surfaces is the flexible, basilar membrane. The basilar
membrane is so termed because it forms part of the floor of the cochlear duct,
thereby differentiating the middle and lower chambers. This membrane, which
von Bekesy discovered is under no tension whatever, is displaced up and down
by the forces exerted on it from beneath. As mentioned earlier, this displacement
ultimately causes a shearing force to be exerted on the hair cell receptors
which are situated in the cochlear duct between the basilar membrane and the
rigid tectorial membrane above them. As can be seen from Figs. 2 and 4, this "receptor
structure" occupies only a small part of the middle cochlear chamber.
Endolymph fills the rest performing a vibration-isolating function. The basilar
membrane, tectorial membrane, and receptor cells in between, which form the
receptor structure, are usually called the organ of Corti. All this may appear
to be an unusually complex system to transfer vibrations to cells which can
respond to them, but in fact, it's elegant simplicity becomes increasingly
apparent as one learns more about it.
By comparison with the processes involved merely in pitch discrimination,
things are simple indeed. A concluding fact or two may provide an adequate
perspective.
The human eardrum has an area of about 70 sq. mm. The area of the oval window
to which vibrations are finally transmitted is only about 3 sq. mm.
Despite this small area, the approximate minimal energy level of a 1000 Hz
tone that we can sense is 0.0002 dyn/cm2 (dyne=energy to accelerate 1 gm at
1 cm/sect). Were our hearing any more sensitive, we would be distracted continuously
by molecular bombardment of our eardrums. In a manner of speaking, then, we
could hear differences in temperature.
The prodigious accomplishment already described is, however, only a fraction
of the story. For we can discriminate identical and different tones of subtly
different sound intensities, perhaps 250 distinct intensity levels. We are
able to make numerous pitch discriminations-about 1,000 of them within the
range of 20 to 16,000 Hz. Moreover, we are sensitive to the "mass" or "volume" of
sound as distinct from loudness; and finally, some of us can make pitch discriminations
of such accuracy that these persons have been said to have perfect (!) pitch.
These phonomena, observed and studied in the psychophysics of audition, deserve
our attention. Yet, we have one more step to consider first-the generation
of neural impulses and transmission of these to the appropriate regions of
the brain.
The Inner Ear
Properly speaking, the hair cells are transducers, not transformers. Up until
this point in the sequence, the energy has been mechanical. Once the hair cells
respond, however, we are no longer dealing with mechanical energy. The hair
cells have generated an electrical potential which neurally represents the
mechanical energy transmitted to them. This potential, often called a generator
potential, is one of several potentials that can be recorded from the inner
ear. The actual mechanisms for the transduction process are not yet known.
Despite that, our knowledge concerning auditory mechanisms has been advanced,
and we can profitably consider the cochlear mechanisms for loudness discrimination
and pitch discrimination. The organ of Corti concerns itself with other auditory
dimensions in addition to these. Perusal of these two processes will demonstrate
the ingenious evolutionary solutions that enable us to perceive extraordinarily
subtle auditory differences in loudness, pitch and a combination of the two.
The Organ of Corti
The greater the vibratory motion of the basilar membrane, the more hair cells
it stimulates to "fire." The more hair cells that fire, the louder
will be the perceived sound. As is often the case, this straight-forward relation
oversimplifies the actual state of affairs. Suffice it to say that the outermost
column of hair cells (which are arrayed in four columns as seen in Fig. 4)
is more receptive to vibration amplitude differences than any of the other
columns. They derive this enhanced sensitivity to amplitude from their physical
position and unique neural connections. These hair cells appear to provide
us with most of our loudness information. Obviously, the loudness information
is in addition to though not necessarily independent of pitch information.
In fact, as many know, these two dimensions of auditory experience are closely
interrelated.
Pitch discrimination is partly a static and partly a dynamic process. The
untensioned basilar membrane is wider at the apex of the cochlear coil than
it is at the base where the coil originates. From Fig. 2, we might expect the
opposite, that the basilar membrane narrows as the coil it's in narrows. Since
the mass of the basilar membrane is least at the base (where it's narrowest),
it's natural vibration frequency is higher there and it is most sensitive to
high frequency vibrations in that region. Conversely, the greater mass of the
basilar membrane at the apex of the cochlear coil renders that area more sensitive
to vibrations of low frequency. Clearly the anatomical shape of the basilar
membrane predisposes it to vibrate maximally at different regions along its
length because, as it goes from base to apex, it gets wider and wider. Additionally,
the higher the frequency, the more localized the area of vibration; very low
frequencies (below 50 Hz) induce the entire membrane to vibrate as a whole.
The waves set up in the basilar membrane are not standing waves from one side
of the membrane to the other (piano string vibration), as had been theorized
a century ago by Hermann von Helmholtz. Dr. von Bekesy has shown that, because
the basilar membrane is not under tension, it vibrates similar to a rope, tied
at one end and waved up and down at the other. This kind of vibratory motion
is a travelling wave. The envelope of a travelling wave will show maximum vibration
amplitude at various regions along an unstretched membrane as a function of
the frequency of vibration and the physical qualities of the membrane. This
characteristic is shown in Fig. 5. While the upper limit of this "tonotopic" organization
and the frequency discrimination deriving from it is not known, it is clear
that this static mechanism contributes greatly to our abilities to discriminate
pitch.
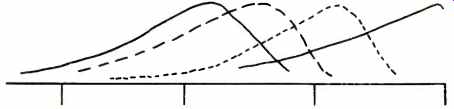
Fig. 5--Travelling waves of different frequencies on the basilar membrane (after von Bekesy).
The second pitch discrimination mechanism that operates in the organ of Corti
is a dynamic, neural one.
In recent experiments, electrodes about a millionth of a meter in diameter
(microelectrodes) have been placed in individual cells of the auditory nerve
or at other auditory central nervous system locations. Research with these "microelectrodes" has shown that special nerve fibers to (not from) the organ of Corti inhibit the
response of hair cells immediately above and below the region of maximum basilar
membrane movement.
That is, these special fibers make it more difficult for hair cells situated
to either side of the maximally stimulated hair cells to respond. The effect
is to increase or "sharpen" the discriminability of one frequency
from another. Of additional importance here is the anatomical fact that all
inner hair cells (Fig. 4), which are known to be primarily involved in pitch
discrimination, are connected to their own individual neural fiber going to
the central nervous system. Thus, precise, detailed information regarding pitch
is transmitted to the higher neural centers.
Not surprisingly, much more happens to the neural signals that traverse these
25,000 or so fibers once they enter the central nervous system, for elegant
though the preceding mechanisms are, they are not sufficient to account for
the incredible pitch discrimination many people show. One of the mechanisms
which occurs inside the central nervous system that is known to increase our
pitch discrimination is the following.
Neuro-physiological research with microelectrodes has established that frequency
discrimination takes place at various levels of the neural circuitry as the
impulses proceed to the portions of the brain that ultimately process and analyze
the auditory impulses. Inside the brain, but not at the highest (cortical)
level, neural inhibitory mechanisms appear to reduce further the neural response
to random (aperiodic) input and to further accentuate a specific response.
All this occurs in a system which ordinarily does not "follow" or
keep track with stimulus frequencies beyond 200 Hz.
This account is necessarily brief.
Nevertheless, it is hoped that some idea of auditory system origin, development,
and functioning has been derived from it. The elegance of this system is surely
obvious. What should also be apparent is that a great deal of signal processing
has taken place even before the signal reaches its ultimate destination, the
auditory regions of the cortex of the brain. A discussion of the processes
occurring in the several brain areas concerned with audition would provide
further insight into the psychology of audition. That enterprise, connecting
the physiology and psychology of hearing, transcends the present topic in complexity
and requires a separate treatment.
References
1. Green, D.M. Audition. Annual Review of Psychology, 20: 105-128, 1969.
2. Milner, P.M. Physiological Psychology. New York: Holt, Rinehart and Winston,
1970.
3. Minkowski, A. (ed.) Regional Development of the Brain in Early Life. Philadelphia,
1967.
4. Ochs, S. Elements of Neurophysiology. New York: John Wiley & Sons,
1965.
5. Peele, T.L. Neuroanatomic Basis for Clinical Neurology. New York: J. Wiley
and Sons, 1957.
6. Peiper, A. Cerebral Function in Infancy and Childhood. New York: Consultants
Bureau, 1963.
7. Romer, A.S. The Vertebrate Body. New York: W.B. Saunders, 1956.
8. Stevens, S.S. (ed.) Handbook of Experimental Psychology, New York: Wiley
and Sons, 1957.
9. von Bekesy, G. Traveling waves as frequency analyzers in the cochlea. Nature,
225; 1207-1209, 1970.
10. Zemlin W.R. Speech and Hearing Science. Englewood Cliffs: Prentice-Hall,
1968.
(Source: Audio magazine, May 1977; Dr. A. Joseph Ray, Jr., Ph.D.*
[Professor of Psychology, East Stroudsburg State College, East Stroudsburg,
Penna. 18301, USA])
= = = =
|