<< cont from part 1
THE SUPERHET SYSTEM
It will have been appreciated from the above that any multiple tuned circuit,
or SAW filter, system chosen to give good selectivity will be optimized only
for one frequency. To tune a group of critically coupled bandpass tuned circuits
simultaneously to cover a required receiver frequency range would be very difficult.
To tune one or more SAW filters simultaneously would simply not be possible
at all, except, perhaps, over an exceedingly limited frequency range.
A practical solution to this problem was offered in 1918 by Major Armstrong
of the US Army, in the form of the supersonic heterodyne or superhet receiver
system, shown in FIG. 21.
In this, the incoming radio signal is combined with an adjustable frequency
local oscillator signal in some element having a non-linear input/output transfer
characteristic, (ideally one having a square-law slope). This stage is known
as the frequency changer or mixer or, sometimes, and inappropriately, as the
first detector.
This mixture of the two (input and LO) signals gives rise to additional sum
and difference frequency outputs, and if the local oscillator frequency is
chosen correctly, one or other of these can be made to coincide with the fixed
intermediate frequency (usually known as the IF), at which the bulk of the
amplification will occur.
The advantages of this arrangement, in allowing the designer to tailor his
selectivity characteristics without regard to the input frequency, are enormous,
and virtually all commercial radio receivers employ one or other of the possible
permutations of this system.
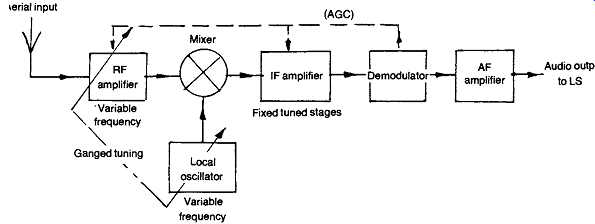
FIG. 21 The superhet system.
PROBLEM
The snag is that the non-linear mixer stage produces both sum and difference
frequency outputs, so that , for any given local oscillator frequency there
will be two incoming signal frequencies at which reception would be possible.
These are known as signal and image frequencies, and it’s essential that the
selectivity of the tuned circuits preceding the mixer stage is adequate to
reject these spurious second channel or image frequency signals. This can be
difficult if the IF frequency is too low in relation to the incoming signal
frequency, since the image frequency will occur at twice the IF frequency removed
from the signal, which may not be a very large proportion of the input signal
frequency, bearing in mind the selectivity limitations of conventional RF tuned
circuits.
In communications receivers and similar high-quality professional systems,
the problem of image breakthrough is solved by the use of the double superhet
system shown in FIG. 22. In this the incoming signal frequency is changed twice,
firstly to a value which is sufficiently high to allow complete elimination
of any spurious image frequency signals, and then, at a later stage, to a lower
frequency at which bandwidth limitation and filtering can be done more easily.
A difficulty inherent in the superhet is that, because the mixer stage is,
by definition, non-linear in its characteristics, it can cause intermodulation
products between incoming signals to be generated, which will be amplified
by the IF stages if they are at IF frequency, or will simply be combined with
the incoming signal if they are large enough to drive the input of the mixer
into overload.
This type of problem is lessened if the mixer device has a true square law
characteristic. Junction FETs have the best performance in this respect.
A further problem is that the mixer stages tend to introduce a larger amount
of noise into the signal chain than the other, more linear, gain stages, and
this may well limit the ultimate sensitivity of the system. The noise figure
of the mixer stage is partly a function of the kind of device or circuit configuration
employed, and partly due to noise introduced by the relatively large amplitude
local oscillator signal.
It’s undoubtedly true to say that the quality and care in design of the mixer
stage from a major determining factor in receiver performance, in respect of
S/N ratio and freedom from spurious signals.
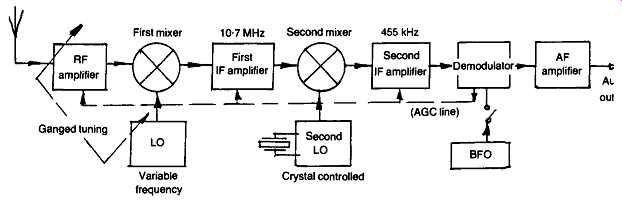
FIG. 22 The double-superhet system, as used in a communication receiver.
OTHER POSSIBILITIES
Because of the problems of the superhet system, in respect of mixer noise,
and image channel interference, direct conversion systems have been proposed,
in which the signal is demodulated by an electronic switch operated by a control
waveform which is synchronous in frequency and phase with the input signal.
These are known as homodyne or synchrodyne receivers, depending on whether
the control waveform is derived from the carrier of the incoming signal or
from a separate synchronous oscillator.
Since both of these systems result in an audio signal in which adjacent channel
transmissions are reproduced at audio frequencies dependent on the difference
of the incoming signal from the control frequency, they offer a means for the
control of selectivity, with a truly flat-topped frequency response, by means
of post-demodulator AF filtering. On the debit side, neither of these offer
the sensitivity or the ease of operation of the conventional superhet, and
are not used commercially to any significant extent.
Sensitivity
Many factors influence this characteristic. The most important of these are:
• the S/N ratio, as depending on the design, small signals can get buried
in mixer or other circuit noise;
• inadequate detectability, due to insufficient gain preceding the demodulator
stage;
• intermodulation effects in which, due to poor RF selectivity or unsatisfactory
mixer characteristics, the wanted signal is swamped by more powerful signals
on adjacent channels.
The ultimate limitation on sensitivity will be imposed by aerial noise, due
either to man-made interference, (RFI), or to the thermal radio emission background
of free space. The only help in this case is a better aerial design.
As mentioned above, the frequency changer stage in a superhet is a weak link
in the receiver chain, as it introduces a disproportionately high amount of
noise, and is prone to intermodulation effects if the signals present exceed
its optimum input signal levels.
The problem of mixer noise is a major one with equipment using thermionic
valves, but semiconductor devices offer substantial improvements in this respect.
For professional equipment, diode ring balanced modulator layouts, using hot
carrier or Schottky diodes, of the kind shown in FIG. 23, are the preferred
choice, since they combine excellent noise characteristics with the best possible
overload margin.
However, this is a complex system.
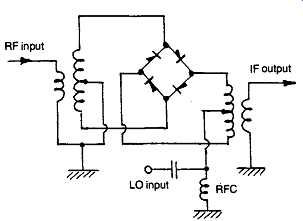
FIG. 23 Diode ring double balanced mixer system.
In domestic equipment, at frequencies up to about 100 MHz, junction FETs are
the preferred devices, though they have inconveniently large inter-electrode
capacitances for RF use. In the frequency range 100-500 MHz, dual-gate MOSFETs
are preferable because their form of construction allows very good input -
output screening, though their noise figure and other characteristics are somewhat
less good than those of junction FETs.
Beyond 500 MHz, bipolar junction transistors are the only practical choice,
though their use demands careful circuit design.
Integrated circuit balanced modulator systems have attracted some interest
for high-quality designs, and have even been proposed as an alternative to
ring diode modulators, though they have relatively poor overload characteristics.
The various practical mixer systems are examined later, under 'Circuit design'.
In general, the best performance in a receiver, in respect of S/N ratio, sensitivity,
and overload characteristics, requires a careful balance between the gain and
selectivity of the various amplifying and mixing stages.
Stability
In a superhet system, in which there are a series of selective fixed frequency
amplifier stages, the major problems of frequency stability center around the
performance of the local (heterodyne) oscillator, which is combined with the
incoming signal to give IF frequency. In relatively narrow bandwidth AM receivers,
especially those operating in the short wave (3-30 MHz) region, a simple valve
or transistor oscillator is unlikely to be adequately stable, unless very good
quality components, and carefully balanced thermal compensation is employed.
Various techniques have been used to overcome this difficulty. For fixed frequency
reception, oscillators based on individual quartz crystal or SAW resonators
- which give an extremely stable output frequency, combined with high purity
and low associated noise - are an excellent solution, though expensive if many
reception frequencies are required.
Alternatively, various ways of taking advantage of the excellent stability
of the quartz crystal oscillator, while still allowing frequency variability,
have been proposed, such as the phase-locked loop (PLL) frequency synthesizer,
or the Barlow-Wadley loop systems.
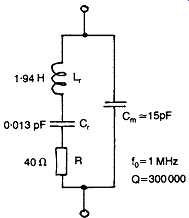
FIG. 24 Equivalent electrical circuit of a quartz crystal resonator.
QUARTZ CRYSTAL CONTROL
This operates by exciting a mechanical oscillation in a precisely dimensioned
slab of mono-crystalline silica, either naturally occurring, as quartz, or,
more commonly synthetically grown from an aqueous solution under conditions
of very high temperature and pressure.
Since quartz exhibits piezo-electric characteristics, an applied alternating
voltage at the correct frequency will cause the quartz slab to 'ring' in a
manner analogous to that of a slab or rod of metal struck by a hammer.
However, in the case of the crystal oscillator, electronic means can be used
to sustain the oscillation.
The monotonic frequency characteristics of the quartz crystal resonator derive
from its very high effective Q value. Typical apparent values of Lr, Cr and
Cm (the resonant inductance and capacitance, and that due to the mounting),
and series loss resistance Ru for an 'X' cut crystal, are shown in the equivalent
circuit of FIG. 24 , for a crystal having a resonant frequency of 1 MHz, and
an effective ß, as a series resonant circuit, of 300000.
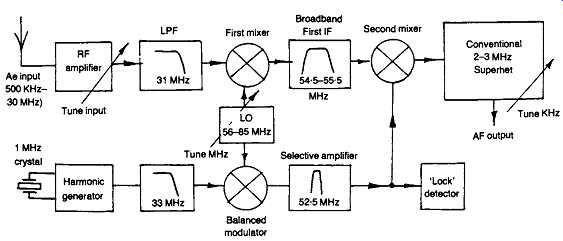
FIG. 25 The Barlow-Wadley loop.
As in other materials, the physical dimensions of quartz crystals will change
with temperature, but since its expansion is anisotropy (greater in some dimensions
than others) it’s possible to choose a particular section, or 'cut', through
the crystal to minimize the effects of temperature on resonant frequency. Such
crystals are known as zero temperature coefficient (ZTC) or AT cut types.
Zero temperature coefficient crystals (in practice this will imply temperature
coefficients of less than ±2 parts per million per degree centigrade over the
temperature range 5-45° C) don’t offer quite such high Q values as those optimized
for this quality, so external temperature stabilization circuits (known as
crystal ovens) are sometimes used in critical applications. Both of these approaches
may also be used, simultaneously, where very high stability is required.
Although, in principle, the quartz crystal is a fixed frequency device, it’s
possible to vary the resonant frequency by a small amount by altering the value
of an externally connected parallel capacitor. This would be quite insufficient
as a receiver tuning means, so other techniques have been evolved.
THE BARLOW-WADLEY LOOP
This circuit arrangement, also known as a drift cancelling oscillator, has
been used for many years in relatively inexpensive amateur communications receivers,
such as the Yaesu Musen FRG-7, and the layout adopted for such a 500 kHz-30
MHz receiver is shown in FIG. 25.
In this, the incoming signal, after appropriate RF amplification and pre-selection,
is passed though a steep-cut, low-pass filter, which removes all signals above
31 MHz, to the first mixer stage. This has a conventional L-C type tuned oscillator
whose operating frequency gives a first IF output in the range 54.5-55.5 MHz,
which is fed to a second mixer stage.
The L-C oscillator output is also fed to a double-balanced modulator where
it’s combined with the output from 1 MHz quartz-crystal controlled harmonic
generator, and this composite output is taken through a selective amplifier
having a center frequency of 52.5 MHz, and a signal output detector system.
Certain combinations of the local oscillator frequency and the harmonics of
1 MHz will be within the required frequency range, and will therefore pass
through this amplifier. When such a condition exists, the output voltage operates
an indicator to show that this signal is present. This output signal is then
fed to the second mixer stage to generate a second IF frequency in the range
2-3 MHz, from which the desired signal is selected by a conventional superhet
receiver from the 1 MHz slab of signals presented to it.
The frequency drift in the first, high-frequency, L-C local oscillator is
thereby cancelled, since it will appear, simultaneously, and in the same sense,
at both the first and second mixers.
FREQUENCY SYNTHESIZER TECHNIQUES
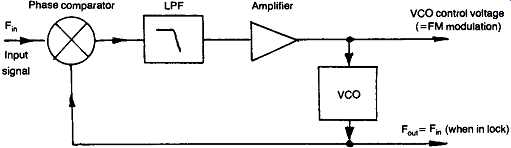
FIG. 26 The phase-locked loop (PLL).
These are based on developments of the phase-locked loop (PLL) shown in FIG.
26. In this arrangement an input AC signal is compared in phase with the output
from a voltage controlled oscillator (VCO). The output from the phase comparator
will be the sum and difference frequencies of the input and VCO signals.
Where the difference frequency is low enough to pass the low-pass 'loop filter',
the resultant control voltage applied to the VCO will tend to pull it into
frequency synchronism, and phase quadrature, with the incoming signal - as
long as the loop gain is high enough. In this condition, the loop is said to
be 'locked'. This circuit can be used to generate an AC signal in frequency
synchronism with, but much larger in amplitude than, the incoming reference
signal. It can also generate an oscillator control voltage which will accurately
follow incoming variations in input signal frequency when the loop is in lock,
and this provides an excellent method of extracting the modulation from an
FM signal.
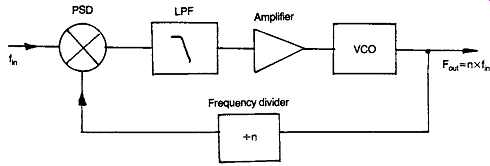
FIG. 27 Phase-locked frequency multiplier.
A further development of the basic PLL circuit is shown in FIG. 27.
In this, a frequency divider is interposed between the VCO and the phase comparator,
so that when the loop is in lock, the VCO output frequency will be a multiple
of the incoming frequency. For example, if the divider has a factor n, then
the VCO will have an output frequency equal to n (Fin). In the PLL frequency
synthesizer shown in FIG. 28, this process is taken one stage further, with
a crystal controlled oscillator as the reference source, feeding the phase
detector through a further frequency divider. If the two dividers have ratios
of m and n, then when the loop is in lock, the output frequency will be (n/m)
x Fref.
Provided that m and n are sufficiently large, the VCO output can be held to
the chosen frequency, with crystal-controlled stability, and with any degree
of precision required. Now that such frequency synthesizer circuitry is available
in single IC form, this type of frequency control is beginning to appear in
high quality FM tuners, as well as in communications receivers.
A minor operating snag with this type of system is that, because of the presence
within the synthesizer IC of very many, relatively large amplitude, switching
waveforms, covering a wide spectrum of frequencies, such receivers tend to
be plagued by a multitude of minor tuning whistles, from which conventional
single tuned oscillator systems are free. Very thorough screening of the synthesizer
chip is necessary to keep these spurious signals down to an unobtrusive level.
Generally, in FM tuners, the relatively wide reception bandwidth makes oscillator
frequency drift a less acute problem than in the case of AM receivers operating
at comparable signal frequencies, although, since the distortion of the received
signals will in most cases deteriorate if the set is not correctly tuned, or
if it drifts off tune during use, there is still an incentive, in high-quality
tuners, to employ quartz crystal stabilized oscillator systems.
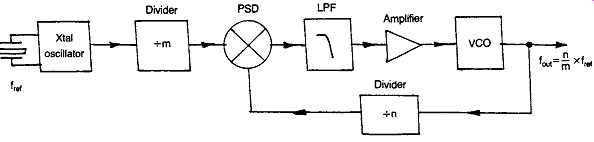
FIG. 28 Phase-locked frequency synthesizer.
A more serious problem, even in wide bandwidth FM tuners -- where these don’t
employ PLL frequency control -- is that the varicap diodes (semiconductor junction
diodes in which the effective capacitance is an inverse function of the applied
reverse voltage) often used to tune the RF and oscillator circuits, are quite
strongly temperature dependant in their characteristics.
Varicap-tuned receivers must therefore employ thermally compensated DC voltage
sources for their tuning controls if drift due to this cause is to be avoided.
AGC EFFECTS
A particular problem which can occur in any system in which automatic gain
control (AGC) is used, is that the operation of the AGC circuit may cause a
sympathetic drift in tuned frequency.
This arises because the AGC system operates by extracting a DC voltage which
is directly related to the signal strength, at some point in the receiver where
this voltage will be of adequate size. This voltage is then used to control
the gain of preceding RF or IF amplifier stages so that the output of the amplifier
remains substantially constant. This is usually done by applying the control
voltage to one or other of the electrodes of the amplifying device so that
its gain characteristics are modified.
Unfortunately, the application of a gain control voltage to any active device
usually results in changes to the input, output, or internal feedback capacitances
of the device, which can affect the resonant frequency of any tuned circuits
attached to it. This effect can be minimized by care in the circuit design.
A different type of problem concerns the time-constants employed in the system.
For effective response to rapid changes in the signal strength of the incoming
signal, the integrating time constant of the system should be as short as practicable.
However, if there is too little constraint on the speed of response of the
AGC system, it may interpret a low-frequency modulation of the carrier, as
For example in an organ pedal note, as a fluctuation in the received signal
strength, and respond accordingly by increasing or decreasing the receiver
gain to smooth this fluctuation out.
In general, a compromise is attempted between the speed of the AGC response,
and the lowest anticipated modulation frequency which the receiver is expected
to reproduce, usually set at 30 Hz in good quality receivers. In transistor
portable radios, where the small LS units seldom reproduce tones below some
200-250 Hz, a much more rapid speed of response is usable without audible tonal
penalties.
Sadly, some broadcasting authorities take advantage of the rapid AGC response
typically found in transistor portables to employ a measure of companding (tonal
range compression on transmission followed by expansion on reception to restore
the original dynamic range). As racticed by the BBC on its Radio 1, Radio
2, Radio 4 and some local radio transmissions, this consists of a reduction
in both carrier strength and modulation on all dynamic peaks. This reduces
the amount of electricity consumed by the transmitter, whose average power
output decreases on sound level peaks.
If, then, the AGC system in the radio restores the received carrier level
to a constant value, the original modulation range will be recovered. This
will only work well if the AGC 'attack' and 'decay' time constants used in
the receiver are correctly related to those employed at the transmitter --
and this is purely a matter of chance. The result, therefore, is an additional
and unexpected source of degradation of the broadcast quality of these signals.
AUTOMATIC FREQUENCY CONTROL (AFC)
Because of the shape of the output voltage versus input frequency relationship,
at the output of the demodulator of an FM receiver, shown in idealized form
in FIG. 29, the possibility exists that this voltage, when averaged so that
it’s just a DC signal, with no carrier modulation, can be used to control the
operating frequency of the oscillator, or other tuned circuits. So if the tuning
of the receiver drifts away from the ideal mid-point (Ft in FIG. 29) an automatic
correction can be applied to restore it to the desired value.
This technique is widely used, especially in the case of receivers where the
tuning is performed by the application of a voltage to one or more varicap
diodes, and which, in consequence, lends itself well to the super imposition
of an additional AFC voltage. It does, however, have snags.
The first of these is that there is a close similarity in the action of an
AFC voltage in an FM tuner to the action of an automatic gain control in an
AM one. In both cases the control system sees the change - in carrier frequency
in the case of an FM tuner - which is produced by the program modulation, as
being an error of the type which the control system is designed to correct.
Too effective an AFC system can therefore lead to a worsening of bass response
in the tuner, unless very heavy damping of the response is incorporated.
In the case of FM tuners, it’s likely that the changes to be corrected will
mainly be slow, and due only to thermal effects, provided that the receiver
was correctly tuned in the first place, whereas in an AM radio the changes
in received signal strength can often be quite rapid.
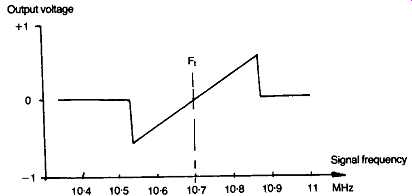
FIG. 29 Voltage/frequency relationships in an ideal FM demodulator.
The second problem is that the AFC system, attempting to sit the tuning point
on the zero DC level, may not lead to the best results if the demodulator circuit
is misaligned, and gives the kind of curve shown in FIG. 30. Here the user
might place the tuning at the point F by ear, where the AFC signal will always
restore it to F_t.
This kind of demodulator misalignment is all too common in practice, and is
one of the reasons why very linear demodulators (in which the choice of the
correct point is less critical) are a worthwhile pursuit. Some more recent
systems employ demodulator circuits which are comparatively insensitive to
detuning. It should be remembered, also, that the output from the demodulator
is likely to be used to operate the tuning meter, and encourage the user to
tune to F_t.
In the case of this kind of tuning meter, the user can carry out a quick check
on the alignment of the tuned circuits by noting the maximum meter deflection
on either side of the incoming signal frequency. These readings should be symmetrical.
Predictability
The possession of a good, clear, tuning scale has always been a desirable
virtue in any radio receiver, so that the user could know the point of tune
and return without difficulty to the same place. However, with contemporary
IC technology, the cost of integrated circuit frequency counter systems has
become so low, relative to the other costs of the design, that almost all modern
tuners now employ some kind of frequency meter display.
This operates in the manner shown in FIG. 31. In this, a quartz crystal controlled
oscillator, operating perhaps at 32.768 kHz, (the standard frequency for 'quartz'
watches , for which cheap crystals and simple frequency dividers are readily
available - 32768 is 215 ) is used to generate a precise time interval, say
one second.
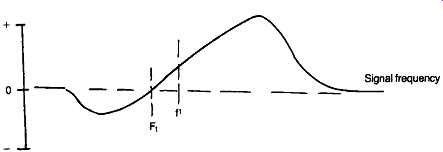
FIG. 30 Effect of demodulator misalignment on AFC operation.
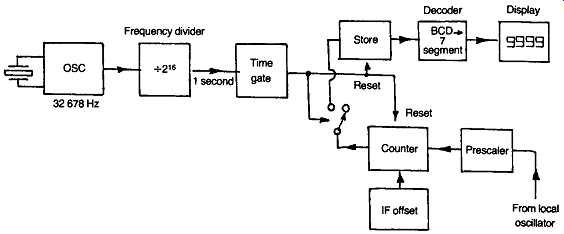
FIG. 31 Frequency meter system.
Meanwhile, the signal from the oscillator of the receiver, which will invariably
employ a superhet layout, will be clocked over this period by an electronic
counter, in which the IF frequency (that frequency by which the local oscillator
differs from the incoming RF signal) will be added to, or subtracted from,
the oscillator frequency - depending on whether the oscillator frequency is
higher or lower than that of the signal - so that the counter effectively registers
the incoming signal frequency.
The electronic switch circuitry operated by the time interval generator is
then used alternately to reset the frequency counter to zero, and to transfer
its final count to a digital frequency store. A liquid crystal (LCD) or light-emitting
diode (LED) display can then be used to register the signal frequency in numerical
form.
As a matter of convenience, to avoid display flicker the counter output will
be held in a store or latch circuit during the counting period, and the contents
of the store only updated each time the count is completed.
Since frequency dither is only likely to affect the last number in the display,
in circumstances where there is an uncertainty of ±1 digit, some systems actually
count to a larger number than is displayed, or up-date the last - least significant
- digit less frequently than the others.
Additional information can also be shown on such an LED/LCD display, such
as the band setting of the receiver, whether it’s on AM or FM, whether AFC
or stereo decoding has been selected, or the time. In the light of the continual
search for marketable improvements, it seems probable that such displays will
soon also include a precise time signal derived from one or other of the time
code transmitters.
Clarity
One of the major advantages of FM radio is its ability to reject external
radio frequency noise - such as that due to thunderstorms, which is very prominent
on LF radio, or motor car ignition noise, which is a nuisance on all HF and
VHF transmissions, which includes Band 2.
This ability to reject such noise depends on the fact that most impulse noise
is primarily amplitude modulated, so that, while it will extend over a very
wide frequency range, its instantaneous frequency distribution is constant.
The design of the FM receiver is chosen, deliberately, so that the demodulator
system is insensitive to AM inputs, and this AM rejection quality is enhanced
by the use of amplitude limiting stages prior to the demodulator.
A valuable ' figure of merit' in an FM receiver is its AM rejection ratio,
and a well-designed receiver should offer at least 60 dB (1000:1). Because
FM receivers are invariably designed so that there is an excess of gain in
the RF and IF stages, so that every signal received which is above the detection
threshold will be presented to the demodulator as an amplitude limited HF square
wave, it’s very seldom that such receivers - other than very exotic and high-cost
designs -- will employ AGC, and only then in the pre-mixer RF stage(s). A further
benefit in well-designed FM receivers is that intruding AM radio signals on
the same band will be ignored, as will less strong FM broadcasts on the same
channel frequency. This latter quality is referred to as the capture ratio,
and is expressed as the difference in the (voltage) signal strength, in dB,
between a more powerful and a less powerful FM transmission, on the same frequency
allocation, which is necessary for the weaker transmission to be ignored.
The capture ratio of an FM receiver - a desirable feature which does not exist
in AM reception - depends on the linearity, both in phase and amplitude, of
the RF and IF stages prior to limiting, and also, to a great extent, on the
type of demodulator employed. The best current designs offer 1 dB discrimination.
Less good designs may only offer 3-4 dB. Two decibels is regarded as an acceptable
figure for high-quality systems, and would imply that the designers had exercised
suitable care.
It should be noted in this context that designs in which very high IF gain
is employed to improve receiver sensitivity may, by causing overload in these
stages, and consequent cross-modulation i f they don’t 'clip' cleanly, lead
to a degradation in capture ratio.
Needless to say, those IF stages which are designed to limit the amplitude
of the incoming signal should be designed so that they don’t generate inter-modulation
products. However, these invariably take the form of highly developed IC designs,
based on circuit layouts of the form shown in FIG. 32, using a sequence of
symmetrically driven, emitter-coupled 'long-tailed pairs', chosen to operate
under conditions where clean and balanced signal clipping will occur.
FM tuner designers very seldom feel inspired to attempt to better their performance
by the use of alternative layouts.
In AM receivers, the best that can be done to limit impulse type interference
is to use some form of impulse amplitude limiter, which operates either on
the detection of the peak amplitude of the incoming signal or on the rate-of-change
of that amplitude, such qualities will be higher on impulse noise than on normal
program content. An excellent AM noise limiter circuit from Philips, which
incorporates an electronic delay line to allow the noise limiter to operate
before the noise pulse arrives, is shown in FIG. 33.
The rejection of intruding signals in AM depends entirely on the receiver
selectivity, its freedom from intermodulation defects, and - in respect of
its ability to avoid internally generated hum, noise, and mush - on the quality
of the circuit design and components used, and the wisdom of the choice of
distribution of the gain and selectivity within the RF and IF stages.
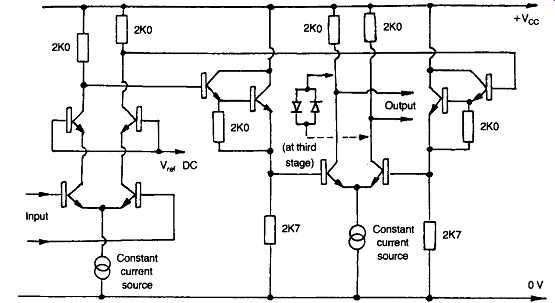
FIG. 32 Cascode input stage, and the first (of two) symmetrical gain stages
in a modern FM IF gain block IC (RCA CA3189E).
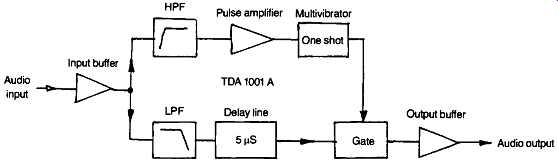
FIG. 33 Philips' impulse noise limiting circuit.
Linearity, FM systems
In view of the continuing search for improved audio amplifier quality in both
quantitative and subjective terms, it’s not surprising that there has been
a comparable effort to obtain a high performance in FM receiver systems.
This endeavor is maintained by competitive rivalry and commercial pressures,
and has resulted in many cases in the development of FM tuners with a quality
which exceeds that of the incoming signal presented to them by the broadcasting
authorities. Broadcasters' activities are not the subject of competitive pressures,
and their standards are determined by the reluctance of governments to spend
money on luxuries, and by the cynicism of their engineers.
These constraints have resulted in a continuing erosion of the quality of
AM radio broadcasts, though there still remain some honorable exceptions. This
has, sadly, often led to the AM sections of FM tuners being designed as low-cost
functional appendages having a very poor audio performance, even when the quality
of the FM section is beyond reproach.
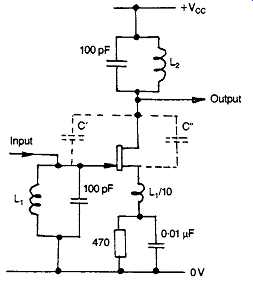
FIG. 34 Feedback neutralization system for junction FET RF amplifier.
A number of factors influence the quality of the demodulated signal, beginning
with the stability and phase linearity of the RF, IF and AF gain stages. In
the case of AM radios, incipient instability in the RF or IF stages will lead
to substantial amplitude distortion effects, with consequent proneness to intermodulation
defects. (Any non-linearity in an amplifier will lead to a muddling of the
signals presented to it.) In the case of those FM radios based on a phase-sensitive
demodulator, any RF or IF instability will lead to substantial phase distortions
as the signal passes through the frequency of incipient oscillation.
Much care is therefore needed in the design of such stages to ensure their
stable operation. If junction FETs are employed rather than dual gate MOSFETs,
some technique, such as that shown in FIG. 34, must be used to neutralize the
residual drain-gate capacitance. The circuit of FIG. 34 utilizes a small inductance
in the source lead, which could be simply a lengthy track on the printed circuit
board, to ensure that the unwanted feedback signal due to the gate-drain capacitance
(C) , is can celled by a signal, effectively in phase opposition, due to the
drain-source capacitance (C"). However, assuming competent RF/IF stage
design, the dominant factor in recovered signal quality is that of the demodulator
design. Representative demodulator systems , for FM and for AM, are shown below.
SLOPE DETECTION
This circuit, shown in FIG. 35(a), is the earliest and crudest method of detecting
or demodulating an FM signal. The receiver is simply tuned to one side or the
other of the tuned circuit resonant frequency (F0), as illustrated in FIG.
35(b). Variations in the incoming frequency will then produce changes in the
output voltage of the receiver, which can be treated as a simple AM signal.
This offers no AM rejection ability, and is very non-linear in its audio response,
due to the shape of the resonance curve.
THE ROUND-TRAVIS DETECTOR
This arrangement, shown in FIG. 36(a), employs a pair of tuned circuits with
associated diode rectifiers ('detectors') which are tuned, respectively, above
and below the incoming signal frequency, giving a balanced slope detector characteristic,
as seen in FIG. 36(b). This is more linear than the simple slope-detector circuit,
but still gives no worthwhile AM rejection.
THE FOSTER-SEELEY OR PHASE DETECTOR
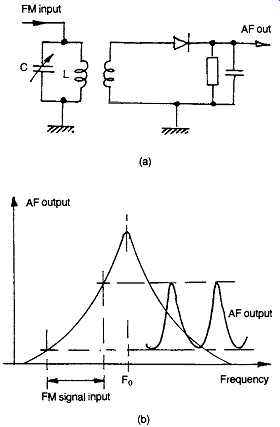
FIG. 35 FM slope detector.
This circuit, of which one form is shown in FIG. 37(a), was evolved to provide
an improved measure of AM rejection, by making its output dependent, at least
in part, on the changes in phase induced in a tuned circuit by variations in
the frequency of the incoming signal.
In this arrangement the tuned circuit, L3Cj, provides a balanced drive to
a matched pair of diode rectifiers (D1 , D2), arranged in opposition so that
any normal AM effects will cancel out. A subsidiary coil, L2, is then arranged
to feed the center tap of L3, so that the induced signal in L2, which will
vary in phase with frequency, will either reinforce or lessen the voltages
induced in each half of L3, by effectively disturbing the position of the electrical
center tap.
This gives the sort of response curve shown in FIG. 37(b) which has better
linearity than its predecessors.
THE RATIO DETECTOR
This circuit, of which one form is shown in FIG. 38(a) is similar to the Foster-Seeley
arrangement, except that the diode rectifiers are connected so that they produce
an output voltage which is opposed, and balanced across the load. The output
response, shown in FIG. 38(b), is very similar to that of the Foster-Seeley
circuit, but it has a greatly improved AM rejection.
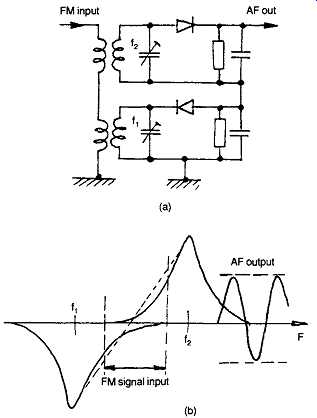
FIG. 36 The Round-Travis FM detector.
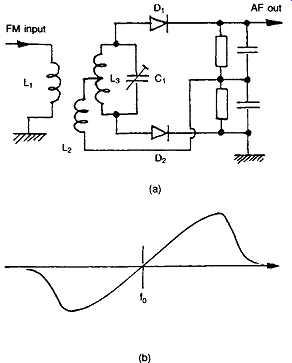
FIG. 37 The Foster-Seeley or phase detector.
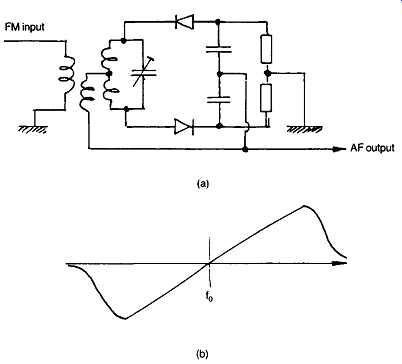
FIG. 38 The ratio detector.
The ratio detector was for many years the basic demodulator circuit for FM
receivers, and offers a very good internal noise figure. This is superior to
that of the contemporary IC-based phase-coincidence system, which has entirely
superseded it, because of the much greater demodulation linearity offered by
this latter system.
THE PHASE COINCIDENCE DEMODULATOR (PCD)
This method employs a circuit layout of the general form shown in FIG. 39,
in which a group of identical bipolar transistors is interconnected so that
the current from Q1, a simple constant-current source, will be routed either
through Q2 or Q3, depending on the relative potential of the signal.
From Q2/Q3, the current flow will be directed either through Q4/Q7 or through
Q5/Q6 and recombined at the load resistors R1 and R2.
It will be seen, from inspection, that if the transistors are well matched,
the potential drop across R1 and R2 will be that due to half the output current
of Q1? regardless of the relative potentials applied to Q2 or Q3 or to Q4/Q7
or Q5/Q6, so long as these potentials are not simultaneously applied.
If synchronous HF signals are applied to all four input ports (a-d), the output
across R1 or R2, (output ports e and f) will only be identical if inputs c
and d are at phase quadrature to those at a and b.
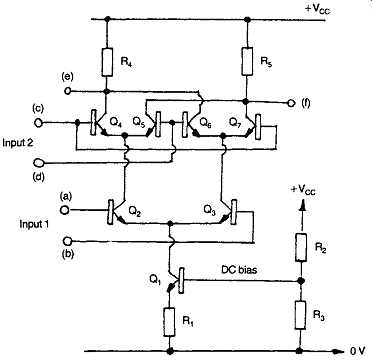
FIG. 39 Gate-coincidence transistor array.
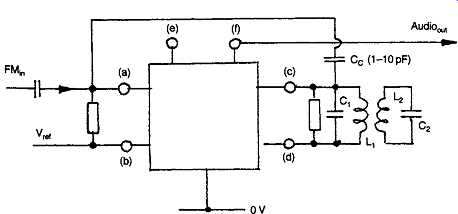
FIG. 40 Phase coincidence demodulator circuit.
Considering the circuit layout of FIG. 40, if ports b and d are taken to some
appropriate DC reference potential, and an amplitude limited FM signal is applied
to point a with respect to b, and some identical frequency signal, at phase
quadrature at F0, is applied to c with respect to d, then there will be an
output voltage at point e with respect to point f, if the input frequency is
varied in respect of F0. The linearity of this VIF relationship is critically
dependent on the short-term frequency/phase stability of the potential developed
across the quadrature circuit (LiQ), and this depends on the Q value of the
tuned circuit. The snag here is that too high a value of Q will limit the usable
FM bandwidth. A normal improvement which is employed is the addition of a further
tuned circuit (L2C2) to give a bandpass coupling characteristic, and further
elaborations of this type are also used to improve the linearity, and lessen
the harmonic distortion introduced by this type of demodulator.
The performance of this type of demodulator is improved if both the signal
drive (to ports a and b) and the feed to the quadrature circuit (ports c and
d) are symmetrical, and this is done in some high quality systems.
THE PHASE-LOCKED LOOP (PLL) DEMODULATOR
This employs a system of the type shown above in FIG. 26. If the voltage controlled
oscillator (VCO) used in the loop has a linear input voltage versus output
frequency characteristic, then when this is in frequency lock with the incoming
signal the control voltage applied to the VCO will be an accurate replica of
the frequency excursions of the input signal, within the limits imposed by
the low-pass loop filter.
This arrangement has a great advantage over all of the preceding demodulator
systems in that it’s sensitive only to the instantaneous input frequency, and
not to the input signal phase. This allows a very low demodulator THD, unaffected
by the phase-linearity of preceding RF and IF tuned circuits or SAW filters,
and greatly reduces the cost , for a given performance standard, of the FM
receiver system.
Such a circuit also has a very high figure for AM rejection and capture ratio,
even when off-tune, provided that the VCO is still in lock.
Photographs of the output signal distortion, and the demodulator output voltage
versus input frequency curves, taken from a frequency modulated oscillator
display, are shown in Figs 41-43 , for actual commercial receivers employing
ratio detector and phase coincidence demodulator systems, together with the
comparable performance of an early experimental PLL receiver due to the author.
All of these units had been in use for some time, and showed the effects of
the misalignment which could be expected to occur with the passage of time.
When new, both the conventional FM tuners would have probably given a better
performance than at the time of the subsequent t test. However, the superiority
of the PLL system is evident.
Commercial manufacturers of domestic style equipment have been slow to exploit
the qualities of the PLL, perhaps deterred by the unpleasant audio signal generated
when the tuner is on the edge of lock. It’s not, though, a difficult matter
to incorporate a muting circuit which cuts off the signal when the receiver
is off tune, and an experimental system of this kind has been in use for many
years.
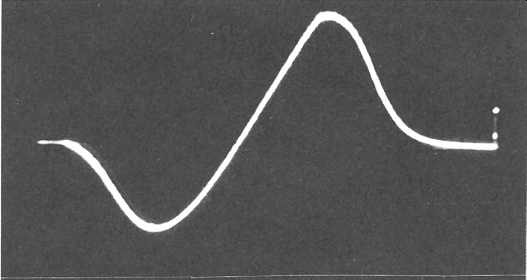
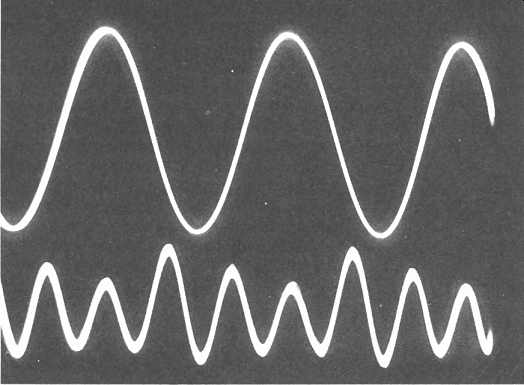
FIG. 41 Practical demodulator frequency/voltage transfer characteristics,
recovered signal, and overall receiver distortion waveform, (THD = 1.5%, mainly
third harmonic) , for ratio detector.
PULSE COUNTING SYSTEMS
In the early years of FM transmissions, when there was great interest in the
exploitation of the very high quality signals then available, pulse counting
systems were commonly employed as the basis for high fidelity amateur designs.
Typical circuit arrangements employed were of the form shown in FIG. 44. After
suitable RF amplification, the incoming signal would be mixed with a crystal
controlled local oscillator signal, in a conventional superhet layout, to give
a relatively low frequency IF in, say, the range 30-180 kHz, which could be
amplified by a conventional broad bandwidth HF amplifier.
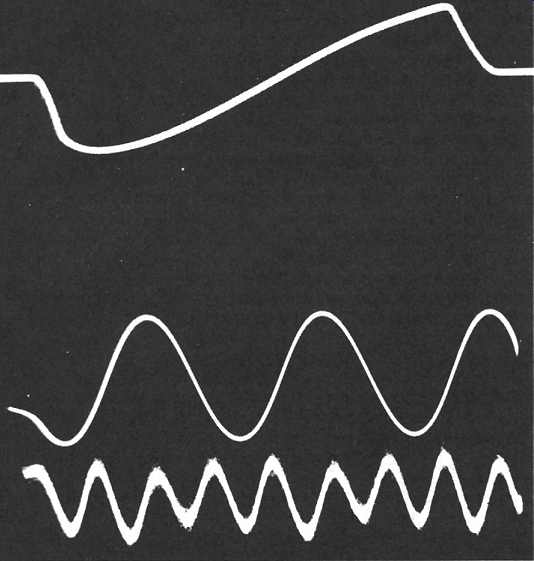
FIG. 42 Practical demodulator frequency/voltage transfer characteristics,
recovered signal, and overall receiver distortion waveform, (THD =0.6%, mainly
third harmonic) , for phase coincidence FM demodulator.
The output signal would then be analyzed by a linear rate meter circuit, to
give a DC output level which was dependent on the instantaneous input signal
frequency, yielding a low distortion recovered AF output.
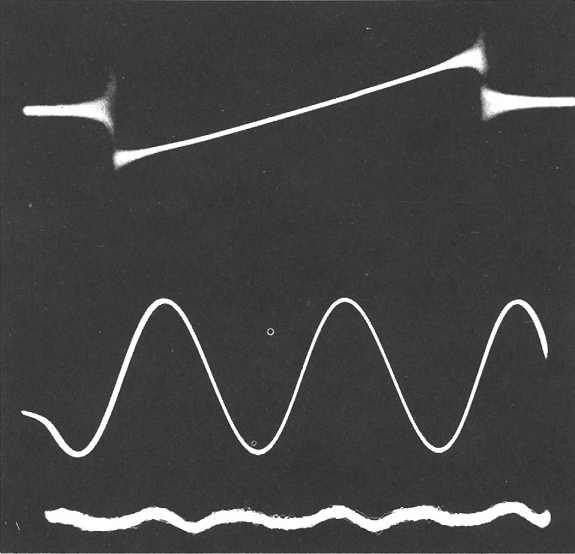
FIG. 43 Practical demodulator frequency I voltage transfer characteristics,
recovered signal, and overall receiver distortion waveform, (THD =0.15%y mainly
second harmonic) , for phase-locked loop FM demodulator.
Such systems shared the quality of the PLL demodulator that the output signal
linearity was largely unaffected by the frequency/phase linearity of the preceding
RF/IF circuitry. Unfortunately, they did not lend themselves well to the demodulation
of stereo signals, and the method fell into disuse.
However, this technique has been resurrected by Pioneer, in its F-90/ F-99
receivers, in a manner which exploits the capabilities of modern digital ICs.
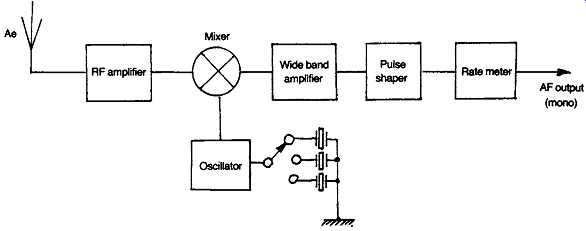
FIG. 44 Pulse counting FM tuner.
The circuit layout employed by Pioneer is shown in schematic form in FIG.
45. In this the incoming 10.7 MHz signal is frequency doubled, to double the
modulation width, and mixed down to 1.26 MHz with a stable crystal controlled
oscillator. After filtering, the signal is cleaned up and converted into a
series of short duration pulses, having constant width and amplitude, of which
the average value is the desired composite (L+R) audio signal.
A conventional PLL arrangement of the type shown in FIG. 27, is then used
to reconstruct the 38 kHz sub-carrier signal, from which the LH and RH stereo
outputs can be derived by adding the (L+R) + (L-R) and (L+R) + (R-L) components.
Practical FM receiver performance data:
The claimed performance from the Pioneer F-90 receiver, using this pulse counting
system, is that the THD is better than 0.01% (mono) and 0.02% (stereo), with
a capture ratio of better than 1 dB, and a stereo channel separation greater
than 60 dB. These figures are substantially better than any obtainable with
more conventional demodulator systems.
In general, the expected THD performance for a good modern FM receiver is
0.1% (mono) and 0.2-0.3% (stereo), with capture ratios in the range 1 dB (excellent)
to 2.5 dB (adequate), and stereo separations in the range 30-50 dB. These figures
largely depend on the type of demodulator employed, and the quality of the
RF and IF circuit components and alignment.
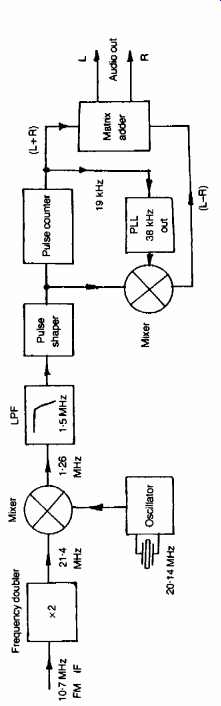
FIG. 45 The Pioneer pulse counting FM tuner system.
Typical AF bandwidths will be in the range 20-40 Hz to 14.5 kHz (-3 dB points).
The LF frequency response depends on the demodulator type, with PLL and pulse
counting systems allowing better LF extension.
The upper frequency limit is set by the requirements of the Zenith-GE encoding
system, rather than by the receiver in use.
The ultimate signal to noise ratio of a good receiver could well be of the
order of 70 dB for a mono signal, but normal reception conditions will reduce
this figure somewhat, to 60 dB or so.
Input (aerial) signal strengths greatly influence the final noise figure,
which worsens at low signal levels, so that input receiver sensitivities of
the order of 2.5 µV and 25 µV might be expected for a 50 dB final S/N figure,
on mono and stereo signals respectively, from a first quality receiver. Values
of 25 µV /100 µV might be expected from less good designs.
A high (aerial) input sensitivity and a good intrinsic receiver S/N ratio
is of value if the receiver is to be used with poor or badly sited aerial systems,
even though the difference between receivers of widely different performance
in this respect may not be noticeable with a better aerial installation.
Linearity, AM systems:
The performance of an AM radio is invariably less good than that possible
from a comparable quality FM receiver. The reasons for this are partly to do
with the greater difficulty in obtaining a low demodulator distortion level
and a wide AF bandwidth, with a sensibly flat frequency response, coupled with
good signal to noise and selectivity figures - a difficulty which is inherent
in the AM system - and partly to do with the quality of the radio signal, which
is often poor, both as received and as transmitted.
There are, however, differences between the various types of AM demodulator,
and some of these have significant performance advantages over those normally
used in practice. These are examined below.
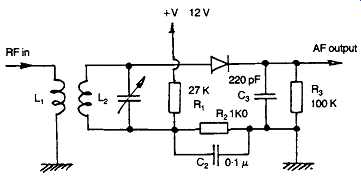
FIG. 46 Simple forward-biased diode AM detector.
THE DIODE 'ENVELOPE' DEMODULATOR
This is a direct descendant of the old crystal detector of the 'crystal and
cat's-whisker' era, and is shown in FIG. 46. In this the peak voltage level
occurring across the tuned circuit L C is passed through the rectifier diode,
??, and stored in the output filter circuit C3R3. Since the rectifying diode
will require some forward voltage to make it conduct (about 0.15 V in the case
of germanium, and 0.55 V in the case of silicon types) it’s good practice to
apply a forward bias voltage, through R!R2C2, to bring the diode to the threshold
of forward conduction.
Because it’s essential that the RF component is removed from the output signal,
there is a minimum practical value for C3. However, since this holds the peak
audio modulation voltage until it can decay through R3, a measure of waveform
distortion is inevitable, especially at higher end of the audio range and at
lower RF input signal frequencies.
Typical performance figures for such demodulators are 1-2% at 1 kHz.
'GRID-LEAK' DEMODULATION
This was a common system used in the days of amateur radio receivers, and
shown in its thermionic valve form in FIG. 47(a). A more modern version of
this arrangement, using a junction FET, is shown in FIG. 47(b). Both these
circuits operate by allowing the RF signal appearing across the tuned circuit
L2Q to develop a reverse bias across R1, which reduces the anode or drain currents.
The THD performance of such circuits is similar to that of the forward biased
diode demodulator, but they have a lower damping effect on the preceding tuned
circuits.
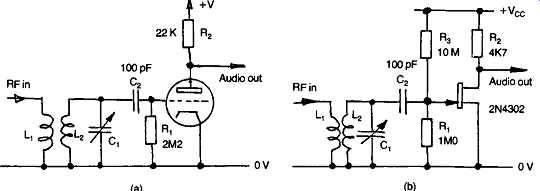
FIG. 47 Valve and FET grid-leak AM detectors.
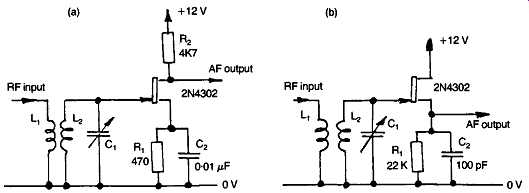
FIG. 48 FET based anode-bend and infinite impedance detectors.
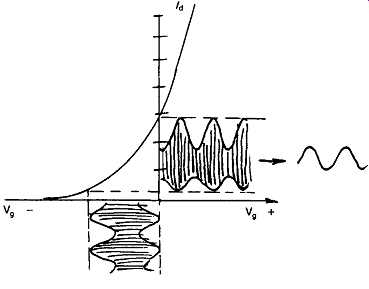
FIG. 49 Method of operation of anode-bend or infinite impedance detectors.
ANODE-BEND OR INFINITE IMPEDANCE DEMODULATOR SYSTEMS
These are shown in their junction FET versions, in Fig 48(a) and 48(b).
They are similar in their action and only differ in the position of the output
load. They operate by taking advantage of the inevitable curvature of the Id/Vg
curve for a valve or FET to provide a distorted version of the incoming radio
signal, as shown in FIG. 49, which, when averaged by some integrating circuit,
gives an audio signal equivalent to the modulation.
Since the circuit distorts the incoming RF waveform, it’s unavoidable that
there will be a related distortion in the recovered AF signal too.
Typical THD figures for such a demodulator system will lie in the range 0.5-1.5%,
depending on signal level. Most IC AM radio systems will employ either diode
envelope detectors or variations of the anode bend arrangement based on semiconductor
devices.
The performance of all these systems can be improved by the addition of an
unmodulated carrier, either at the same frequency, or at one substantially
different from it, to improve the carrier to modulation ratio.
This is not a technique which is used for equipment destined for the domestic
market, in spite of the quality improvement possible.
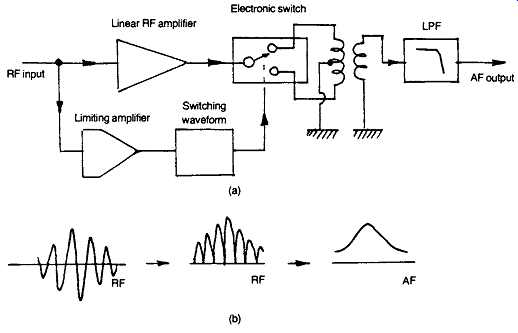
FIG. 50 Circuit layout and method of operation of Homodyne type synchronous
demodulator.
SYNCHRONOUS DEMODULATION
This method, illustrated schematically in FIG. 50(a), operates by synchronously
inverting the phase of alternate halves of the RF signal, so that the two halves
of the modulated carrier can be added together to give a unidirectional output.
Then, when the RF component is removed by filtering, only the audio modulation
waveform remains, as shown in FIG. 50(b). This technique is widely used in
professional communication systems, and is capable of THD values well below
0.1%.
CIRCUIT DESIGN
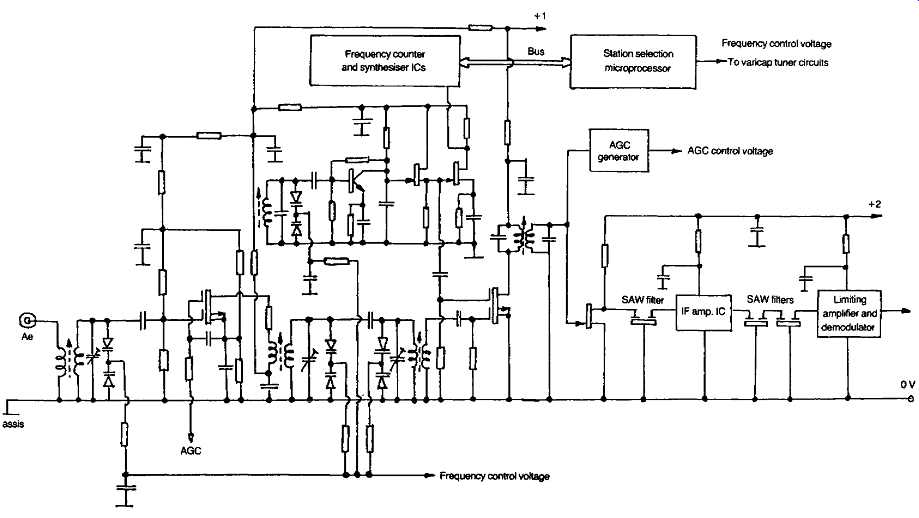
FIG. 51 Typical circuit layout of RF and IF stages of good quality contemporary
FM tuner.
Although there is a continuing interest in circuit and performance improvements
made possible by new circuit and device technology, there is also a tendency
towards a degree of uniformity in circuit design, as certain approaches establish
themselves as the best, or the most cost-effective.
This is particularly true in the RF and IF stages of modern FM tuners, and
an illustration of the type of design approach employed is given in FIG. 51.
The only feature in this not covered by the previous discussion is the general
use of dual (back-to-back) Varicap diodes. These are preferred to single diodes
as the tuning method in high-quality systems because they avoid the distortion
of the RF waveform brought about by the signal voltage modulating the diode
capacitance.
Variable air-spaced ganged capacitors would be better still, but these are
bulky and expensive, and don’t lend themselves to frequency synthesizer layouts.
NEW DEVELOPMENTS
In such a fast changing market it’s difficult to single out recent or projected
design features for special mention, but certain trends are apparent. These
mainly concern the use of microprocessor technology to memorize and store user
selections of channel frequencies, as in the Quad FM4 ( FIG. 52), and the use
of 'sliding stereo separation' systems to lessen the L-R channel separation,
with its associated 'stereo hiss', when the incoming signal strength falls
below the optimum value.
Some synthesizer tuners offer normal spin-wheel tuning, as in the Harmon-Kardon
TU915, by coupling the tuning knob shaft to the synthesizer IC by the use of
an optical shaft encoder.
Low-noise gallium arsenide dual-gate Mosfets have made an appearance in the
Hitachi FT5500 receiver, and these devices are likely to be more widely adopted
in such systems.
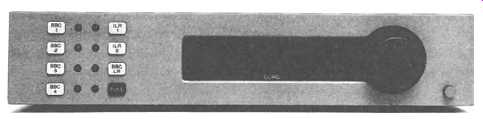
FIG. 52 The Quad FM tuner.
Clearly, commercial pressures will encourage manufacturers to develop more
complex large-scale integration (LSI) integrated circuits, so that more of
the receiver circuitry may be held on a single chip. This undoubtedly saves
manufacturing costs, but the results are not always of benefit to the user,
as evidenced by the current performance of single IC AM radio sections.
An increasing number of the better FM tuners are now offering a choice of
IF bandwidths, to permit user optimization of selectivity, sensitivity or stereo
separation. Variable receiver bandwidth would be a valuable feature on the
MF bands, and may be offered if there is any serious attempt to improve the
quality of this type of receiver.
APPENDIX 1: BROADCAST SIGNAL CHARACTERISTICS
(Data by courtesy of the BBC) Audio bandwidths MF:
• 40-5800 Hz ±3 dB, with very sharp cut off. (> 24 dB/octave beyond 6 kHz.)
• 50-5000 Hz ±1 dB. VHF:
• 30-15,000 Hz ±0.5 dB, with very sharp cut off beyond this frequency.
Distortion MF:
• < 3% THD at 75% modulation.
• < 4% THD at 100% modulation.
VHF:
• < 0.5% THD. Stereo crosstalk VHF: > 46 dB. (0.5%). Modulation depth
MF: Up to 100% over the range 100-5000 Hz.
VHF: Peak deviation level corresponds to ±60.75 kHz deviation.
(The total deviation, including pilot tone, is ±75 kHz.) S/N ratio MF: > 54
dB below 100% modulation.
VHF: Up to 64 dB, using CCIR/468 weighting, with reference to peak program
modulation level.
APPENDIX 2: RADIO DATA SYSTEM (RDS) (Data by courtesy of the BBC)
It was proposed to begin the introduction of this system in the Autumn of
1987, and when in use on receivers adapted to receive this signal the reception
of station, program and other data is possible.
This data will include program identification, to allow the receiver to automatically
locate and select the best available signal carrying the chosen program, and
to display in alphanumeric form a suitable legend, and to permit display of
clock time and date.
Anticipated future developments of this system include a facility for automatic
selection of program type (speech/light music/serious music/ news), and for
the visual display of information, such as traffic conditions, phone-in numbers,
news flashes, program titles or contents, and data print out via a computer
link.
|