<<Prev.
3.5 Preparation
There are many measurements that can be performed on a sound system. A prerequisite
to any measurement is to answer the following questions:
1. What am I trying to measure?
2. Why am I trying to measure it?
3. Is it audible?
4. Is it relevant?
Failure to consider these questions can lead to hours of wasted time and a
hard drive full of meaningless data. Even with the incredible technologies
that we have available to us, the first part of any measurement session is
to listen. It can take many hours to determine what needs to be measured to
solve a sound system problem, yet the actual measurement itself can often be
completed in seconds. Using an analogy from the medical field, the physician
must query the patient at length to narrow down the ailment. The more that
is known about the ailment, the more specific and relevant the tests that can
be run for diagnosis. There is no need to test for tonsillitis if the problem
is a sore back!
1. What am I measuring? A fundamental decision that precedes a meaningful
measurement is how much of the room's response to include in the measured data.
Modern measurement systems have the ability to perform semi-anechoic measurements,
and the measurer must decide if the loudspeaker, the room, or the combination
needs to be measured. If one is diagnosing loudspeaker ailments, there is little
reason to select a time window long enough to include the effects of late reflections
and reverberation. A properly selected time window can isolate the direct field
of the loudspeaker and allow its response to be evaluated independently of
the room.
If one is trying to measure the total decay time of the room, the direct sound
field becomes less important, and a microphone placement and time window are
selected to capture the entire energy decay. Most modern measurement systems
acquire the complete impulse response, including the room decay, so the choice
of the time window size can be made after the fact during post processing.
2. Why am I measuring? There are several reasons for performing acoustic measurements
in a space. An important reason for the system designer is to characterize
the listening environment. Is it dead? Is it live? Is it reverberant? These
questions must be considered prior to the design of a sound system for the
space. While the human hearing system can provide the answers to these questions,
it cannot document them and it is easily deceived. Measurements might also
be performed to document the performance of an existing system prior to performing
changes or adding room treatment.
Customers sometimes forget how bad it once sounded after a new or upgraded
system is in place for a few weeks.
The most common reason for performing measurements on a system is for calibration
purposes. This can include equalization, signal alignment, crossover selection,
and a multiplicity of other reasons. Since loudspeakers interact in a complex
way with their environment, the final phase of any system installation is to
verify system performance by measurement.
3. Is it audible? Can I hear what I am trying to measure? If one cannot hear
an anomaly, there is little reason to attempt to measure it. The human hearing
system is perhaps the best tool available for determining what should be measured
about a sound system. The human hearing system can tell us that something doesn't
sound right, but the cause of the problem can be revealed by measurement. Anything
you can hear can be measured, and once it is measured it can be quantified
and manipulated.
4. Is it relevant? Am I measuring something that is worth measuring? If one
is working for a client, time is money. Measurements must be prioritized to
focus on audible problems. Endless hours can be spent "chasing rabbits" by
measuring details that are of no importance to the client. This is not necessarily
a fruitless process, but it is one that should be done on your own time. I
have on several occasions spent time measuring and documenting anomalies that
had nothing to do with the customer's reason for calling me. All venues have
problems that the owner is unaware of. Communication with the client is the
best way to avoid this pitfall.
3.5.1 Dissecting the Impulse Response
The audio practitioner is often faced with the dilemma of determining whether
the reason for bad sound is the loudspeaker system, the room, or an interaction
of the two. The impulse response can hold that answer to these and other perplexing
questions. The impulse response in its amplitude versus time display is not
particularly useful for other than determining the polarity of a system component,
FIG. 15. A better representation comes from squaring impulse response (making
all deflections positive) and displaying the square root of the result on a
logarithmic vertical scale. This log-squared response allows the relative levels
of energy arrivals to be compared, FIG. 16.
3.5.2 The Envelope-Time Curve
Another useful way of viewing the impulse response is in the form of the envelope-time
curve, or ETC. The ETC is also a contribution of Richard Heyser. It takes the
real part of the impulse response and combines it with a 90 degrees phase shifted
version of the same, FIG. 17. One way to get the shifted version is to use
the Hilbert Transform. The complex combination of these two signals yields
a time domain waveform that is often easier to interpret than the impulse response.
The ETC can be loosely thought of as a smoothing function for the log-squared
response, showing the envelope of the data. This can be more revealing as to
the audibility of an event. The impulse response, log-squared response, and
energy-time curve are all different ways to view the time domain data.
3.5.3 A Global Look
When starting a measurement session, a practical approach is to first take
a global look and measure the complete decay of the room. The measurer can
then choose to ignore part of the time record by using a time window to isolate
the desired part during postprocessing. The length of the time window can be
increased to include the effects of more of the energy returned by the room.
The time window can also be used to isolate a reflection and view its spectral
content. Just like your life span represents a time window in human history,
a time window can be used to isolate parts of the impulse response.
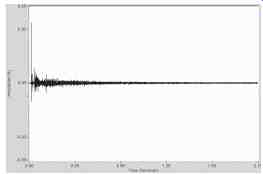 FIG. 15. The impulse response.
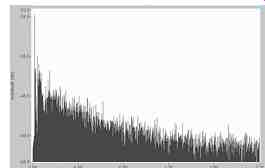 FIG. 16. The log-squared response.
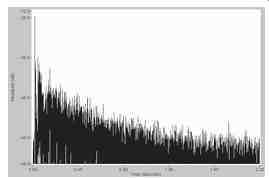 FIG. 17. The envelope-time curve (ETC).
3.5.4 Time Window Length
The time domain response can be divided to identify the portion that can be
attributed to the loudspeaker and that which can be attributed to the room.
It must be emphasized that there is a rather gray and frequency-dependent line
between the two, but for this discussion we will assume that we can clearly
separate them. The direct field is the energy that arrives at the listener
prior to any reflections from the room. The division is fairly distinct if
neither the loudspeaker nor microphone is placed near any reflecting surfaces,
which, by the way, is a good system design practice. At long wavelengths (low
frequencies) the direct field may include the effects of boundaries near the
loudspeaker and micro phone. As frequency increases, the sound from the loud
speaker becomes less affected by boundary effects (due in part to increased
directivity) and can be measured independently of them. Proper loudspeaker
placement produces a time gap between the sound energy arrivals from the loudspeaker
and the later arriving room response. We can use this time gap to aid in selecting
a time window to separate the loudspeaker response from the room response and
diagnosing system problems.
3.5.5 Acoustic Wavelengths
Sound travels in waves. The sound waves that we are interested in characterizing
have a physical size. There will be a minimum time span required to observe
the spectral response of a waveform. The minimum required length of time to
view an acoustical event is determined by the longest wavelength (lowest frequency)
present in the event. At the upper limits of human hearing, the wavelengths
are only a few millimeters in length, but as frequency decreases the waves
become increasingly larger. At the lowest frequencies that humans hear, the
wavelengths are many meters long, and can actually be larger than the listening
(or measurement) space. This makes it difficult to measure low frequencies
from a loudspeaker independently of the listening space, since low frequencies
radiated from a loudspeaker interact (couple) with the surfaces around them.
In an ideally positioned loudspeaker, the first energy arrival from the loudspeaker
at mid- and high frequencies has already dissipated prior to the arrival of
reflections and can therefore often be measured independently of them. The
human hearing system tends to fuse the direct sound from the loudspeaker with
the early reflections from nearby surfaces with regard to level (loudness)
and frequency (tone). It is usually useful to consider them as separate events,
especially since the time offset between the direct sound and first reflections
will be unique for each listening position. This precludes any type of frequency
domain correction (i.e., equalization) of the room/loudspeaker response other
than at frequencies where coupling occurs due to close proximity to nearby
surfaces. While it is possible to compensate to some extent for room reflections
at a point in space (acoustic echo cancellers used for conference systems),
this correction cannot be extended to include an area. This inability to compensate
for the reflected energy at mid/high frequencies suggests that their effects
be removed from the loudspeaker's direct field response prior to meaningful
equalization work by use of an appropriate time window.
3.5.6 Microphone Placement
A microphone is needed to acquire the sound radiated into the space from the
loudspeaker at a discrete position. Proper microphone placement is determined
by the type of test being performed. If one were interested in measuring the
decay time of the room, it is usually best to place the microphone well beyond
critical distance.
This allows the build-up of the reverberant field to be observed as well as
providing good resolution of the decaying tail. Critical distance is the distance
from the loudspeaker at which the direct field level and reverberant field
level are equal. It is described further in Section 3.5.7. If it's the loudspeaker's
response that needs to be measured, then a microphone placement inside of critical
distance will provide better data on some types of analyzers, since the direct
sound field is stronger relative to the later energy returning from the room.
If the microphone is placed too close to the loudspeaker, the measured sound
levels will be accurate for that position, but may not accurately extrapolate
to greater distances with the inverse-square law. As the sound travels farther,
the response at a remote listening position may bear little resemblance to
the response at the near field microphone position. For this reason, it is
usually desirable to place the microphone in the far free field of the loudspeaker-not
too close and not too far away. The approximate extent of the near field can
be determined by considering that the path length difference from the measurement
position (assumed axial) and the edge of the sound radiator should be less
than 1/4 wavelength at the frequency of interest. This condition is easily
met for a small loudspeaker that is radiating low frequencies.
Such devices closely approximate an ideal point source.
As the frequency increases the condition becomes more difficult to satisfy,
especially if the size of the radiator also increases. Large radiators (or
groups of radiators) emitting high frequencies can extend the near field to
very long distances. Line arrays make use of this principle to overcome the
inverse-square law. In practice, small bookshelf loudspeakers can be accurately
measured at a few meters. About 10 m is a common measurement distance for moderate-sized,
full-range loudspeakers in a large space. Even greater distances are required
for large devices radiating high frequencies. A general guideline is to not
put the mic closer than three times the loudspeaker's longest dimension.
3.5.7 Estimate the Critical Distance DC Critical distance is easy to estimate.
A quick method with adequate accuracy requires a sound level meter and noise
source. Ideally, the noise source should be band limited, as critical distance
is frequency dependent. The 2 kHz octave band is a good place to start when
measuring critical distance. Proceed as follows:
1. Energize the room with pink noise in the desired octave band from the sound
source being measured. The level should be at least 25 dB higher than the background
noise in the same octave band.
2. Using the sound level meter, take a reading near the loudspeaker (about
1 m) and on-axis. At this distance, the direct sound field will dominate the
measurement.
3. Move away from the loudspeaker while observing the sound level meter. The
sound level will fall off as you move farther away. If you are in a room with
a reverberant sound field, at some distance the meter reading will quit dropping.
You have now moved beyond critical distance. Measurements of the direct field
beyond this point will be a challenge for some types of analysis. Move back
toward the loudspeaker until the meter begins to rise again. You are now entering
a good region to perform acoustic measurements on loudspeakers in this environment.
The above process provides an estimate that is adequate for positioning a measurement
microphone for loudspeaker testing. With a mic placement inside of critical
distance, the direct field is a more dominant feature on the impulse response
and a time window will be more effective in removing room reflections.
At this point it is interesting to wander around the room with the sound level
meter and evaluate the uniformity of the reverberant field. Rooms that are
reverberant by the classical definition will vary little in sound level beyond
critical distance when energized with a continuous noise spectrum. Such spaces
have low internal sound absorption relative to their volume.
3.5.8 Common Factors to All Measurement Systems
Let's assume that we wish
to measure the impulse response of a loudspeaker/room combination. While
it would not be practical to measure the response at every seat, it is good
measurement practice to measure at as many seats as are required to prove the
performance of the system. Once the impulse response is properly acquired,
any number of post-processes can be per formed on the data to extract information
from it. Most modern measurement systems make use of digital sampling in acquiring
the response of the system. The fundamentals and prerequisites are not unlike
the techniques used to make any digital recording, where one must be concerned
with the level of an event and its time length. Some setup is required and
some fundamentals are as follows:
1. The sampling rate must be fast enough to capture the highest frequency
component of interest. This requires at least two samples of the highest frequency
component. If one wished to measure to 20 kHz, the required sample rate would
need to be at least 40 kHz. Most measurement systems sample at 44.1 kHz or
48 kHz, more than sufficient for acoustic measurements.
2. The time length of the measurement must be long enough to allow the decaying
energy curve to flatten out into the room noise floor. Care must be taken to
not cut off the decaying energy, as this will result in artifacts in the data,
like a scratch on a phonograph record. If the sampling rate is 44.1 kHz, then
44,100 samples must be collected for each second of room decay. A 3-second
room would therefore require 44.1 × 1000 × 3 or 128,000 samples. A hand clap
test is a good way to estimate the decay time of the room and therefore the
required number of samples to fully capture it. The time span of the measurement
also determines the lowest frequency that can be resolved from the measured
data, which is approximately the inverse of the measurement length. The sampling
rate can be reduced to increase the sampling time to yield better low-frequency
information. The trade-off is a reduction in the highest frequency that can
be measured, since the condition outlined in step one may have been violated.
3. The measurement must have a sufficient signal-to noise ratio to allow the
decaying tail to be fully observed. This often requires that the measurement
be repeated a number of times and the results averaged. Using a dual-channel
FFT or MLS, the improvement in SNR will be 3 dB for each doubling of the number
of averages. Ten averages is a good place to start, and this number can be
increased or decreased depending on the environment. The level of the test
stimulus is also important. Higher levels produce improved SNR, but can also
stress the loudspeaker.
4. Perform the test and observe the data. It should fill the screen from top
left to bottom right and be fully decayed prior to reaching the right side
of the screen. It should also be repeatable. Run the test several times to
check for consistency. Background noise can dramatically affect the repeatability
of the measurement and the validity of the data.
Once the impulse response is acquired, it can be further analyzed for spectral
content, intelligibility information, decay time, etc. These are referred to
as metrics, and some require some knowledge on the part of the measurer in
properly placing markers (called cursors) to identify the parameters required
to perform the calculations. Let us look at how the response of the loudspeaker
might be extracted from the data just gathered.
The time domain data displays what would have resulted if an impulse were
fed through the system.
Don't try to correlate what you see on the analyzer with what you heard during
the test. Most measurement systems display an impulse response that is calculated
from a knowledge of the input and output signal to the system, and there is
no resemblance between what you hear when the test is run and what you are
seeing on the screen, FIG. 18.
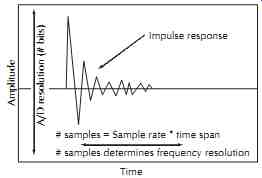 FIG. 18. Many analyzers acquire the room response by digital sampling.
Amplitude Time
# samples = Sample rate * time span
# samples determines frequency resolution A/D resolution (# bits) Impulse
response 1»45
We can usually assume that the first energy arrival is from the loudspeaker
itself, since any reflection would have to arrive later than the first wave
front since it had to travel farther. Pre-arrivals can be caused by the acoustic
wave propagating through a solid object, such as a ceiling or floor and reradiating
near the microphone.
Such arrivals are very rare and usually quite low in level.
In some cases a reflection may actually be louder than the direct arrival.
This could be due to loudspeaker design or its placement relative to the mic
location. It's up to the measurer to determine if this is normal for a given
loudspeaker position/seating position. All loud speakers will have some internal
and external reflections that will arrive just after the first wave front.
These are actually a part of the loudspeaker's response and can't be separated
from the first wave front with a time window due to their close proximity without
extreme compromises in frequency resolution. Such reflections are at least
partially responsible for the characteristic sound of a loudspeaker. Studio
monitor designers and studio control room designers go to great lengths to
reduce the level of such reflections, yielding more accurate sound reproduction.
Good system design practice is to place loudspeakers as far as possible from
boundaries (at least at mid- and high frequencies). This will produce an initial
time gap between the loudspeaker's response and the first reflections from
the room. This gap is a good initial dividing point between the loudspeaker's
response and the room's response, with the energy to the left of the dividing
cursor being the response of the loud speaker and the energy to the right the
response of the room. The placement of this divider can form a time window
by having the analyzer ignore everything later in time than the cursor setting.
The time window size also determines the frequency resolution of the post processed
data. In the frequency domain, improved resolution means a smaller number.
For instance, 10 Hz resolution is better than 40 Hz resolution. Since time
and frequency have an inverse relationship, the time window length required
to observe 10 Hz will be much longer than the time window length required to
resolve 40 Hz.
The resolution can be estimated by f =1/T, where T is the length of the time
window in seconds. Since a frequency magnitude plot is made up of a number
of data points connected by a line, another way to view the frequency resolution
is that it is the number of Hz between the data points in a frequency domain
display.
The method of determination of the time window length varies with different
analyzers. Some allow a cursor to be placed anywhere on the data record, and
the placement determines the frequency resolution of the spectrum determined
by the window length. Others require that the measurer select the number of
samples to be used to form the time window, which in turn deter mines the frequency
resolution of the time window. The window can then be positioned at different
places on the time domain plot to observe the spectral content of the energy
within the window, FIGs. 19, 20, and 21.
For instance, a 1 second total time (44,100 samples) could be divided into
about twenty two time windows of 2048 samples each (about 45 ms). Each window
would allow the observation of the spectral content down to ( ) × 1000 or 22
Hz. The windows can be overlapped and moved around to allow more precise selection
of the time span to be observed. Displaying a number of these time windows
in succession, each separated by a time offset, can form a 3D plot known as
a waterfall.
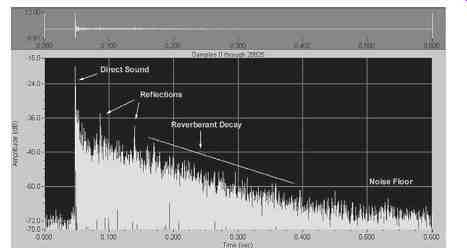 FIG. 19. A room response showing the various sound fields that can exist
in an enclosed space.
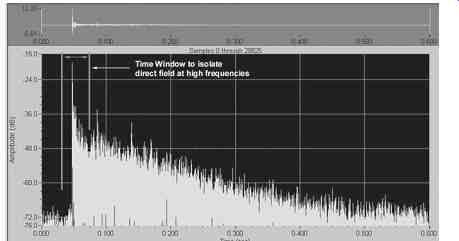
FIG. 20. A time window can be used to isolate the loudspeaker's response
from the room reflections.
3.5.9 Data Windows
There are some conditions that must be observed when placing cursors to define
the time window. Ideally, we would like to place the cursor at a point on the
time record where the energy is zero. A cursor placement that cuts off an energy
arrival will produce a sharp rise or fall time that produces artifacts in the
resultant calculated spectral response. Discontinuities in the time domain
have broad spectral content in the frequency domain. A good example is a scratch
on a phonograph record. The discontinuity formed by the scratch manifests itself
as a broadband click during playback. If an otherwise smooth wheel has a discontinuity
at one point, it would thump annoyingly when it was rolled on a smooth surface.
Our measurement systems treat the data within the selected window as a continuously
repeating event. The end of the event must line up with the beginning or a
discontinuity occurs resulting in the generation of high-frequency artifacts
called spectral leakage. In the same manner that a physical discontinuity in
a phonograph record or wheel can be corrected by polishing, a discontinuity
in a sampled time measurement can be remedied by tapering the energy at the
beginning and end of the window to zero using a mathematical function. A number
of data window shapes are available for performing the smoothing.
These include the Hann, Hamming, Blackman Harris, and others. In the same
way that a physical polishing process removes some good material from what
is being rubbed, data windows remove some good data in the process of smoothing
the discontinuity. Each window has a particular shape that leaves the data
largely untouched at the center of the window but tapers it to varying degrees
toward the edges. Half windows only smooth the data at the right edge of the
time record while full windows taper both (start and stop) edges.
Since all windows have side effects, there is no clear preference as to which
one should be used. The Hann window provides a good compromise between time
record truncation and data preservation. FIGs. 22 and 46-23 show how a data
window might be used to reduce spectral leakage.
3.5.10 A Methodical Approach
Since there are an innumerable number of tests that can be performed on a
system, it makes sense to establish a methodical and logical process for the
measurement session. One such scenario may be as follows:
1. Determine the reason for and scope of the measurement session. What are
you looking for? Can you hear it? Is it repeatable? Why do you need this information?
2. Determine what you are going to measure. Are you looking at the room or
at the sound system? If it is the room, possibly the only meaningful measurements
will be the overall decay time and the noise floor. If you are looking at the
sound system, decide if you need to switch off or disconnect some loudspeakers.
This may be essential to determine whether the individual components are working
properly, or that an anomaly is the result of interaction between several components. "Divide
and conquer" is the axiom.
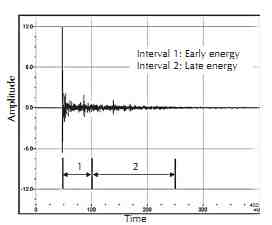 FIG. 21. Increasing the length of the time window increases the frequency
resolution, but lets more of the room into the measurement.
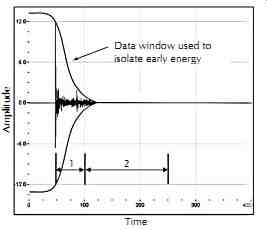 FIG. 22. The impulse response showing both early and late energy arrivals.
3. Select the microphone position. I usually begin by looking at the on-axis
response of the loudspeaker as measured from inside of critical distance. If
multiple loudspeakers are on, turn all of them off but one prior to measuring.
The microphone should be placed in the far free field of the loudspeaker as
previously described. When measuring a loud speaker's response, care should
be taken to eliminate the effects of early reflections on the measured data,
as these will generate acoustic comb filters that can mask the true response
of the loudspeaker.
In most cases the predominant offending surface will be the floor or other
boundaries near the microphone and loudspeaker. These reflections can be reduced
or eliminated by using a ground plane microphone placement, a tall microphone
stand (when the loudspeaker is overhead), or some strategically placed absorption.
I prefer the tall micro phone stand for measuring installed systems with seating
present since it works most anywhere, regardless of the seating type. The idea
is to intercept the sound on its way to a listener position, but before it
can interact with the physical boundaries around that position. These will
always be unique to that particular seat, so it is better to look at the free
field response, as it is the common denominator to many listener seats.
4. Begin with the big picture. Measure an impulse response of the complete
decay of the space. This yields an idea of the overall properties of the room/system
and provides a good point of reference for zooming in to smaller time windows.
Save this information for documentation purposes, as later you may wish to
reopen the file for further processing.
5. Reduce the size of the time window to eliminate room reflections. Remember
that you are trading off frequency resolution when truncating the time record,
FIG. 24. Be certain to maintain sufficient resolution to allow adequate low-frequency
detail.
In some cases, it may be impossible to maintain a sufficiently long window
to view low frequencies and at the same time eliminate the effects of reflections
at higher frequencies, FIG. 25. In such cases, the investigator may wish to
use a short window for looking at the high-frequency direct field, but a longer
window for evaluating the woofer. Windows appropriate for each part of the
spectrum can be used. Some measurement systems provide variable time windows,
which allow low frequencies to be viewed in great detail (long time window)
while still providing a semi-anechoic view (short time window) at high frequencies.
There is evidence to support that this is how humans process sound information,
making this method particularly interesting, FIG. 26.
6. Are other microphone positions necessary to characterize this loudspeaker?
The off-axis response of some loudspeakers is very similar to the on-axis response,
reducing the need to measure at many angles. Other loudspeakers have very erratic
responses, and a measurement at any one point around the loudspeaker may bear
little resemblance to the response at other positions. This is a design issue,
but one that must be considered by the measurer.
7. Once an accurate impulse response is measured, it can be post-processed
to yield information on spectral content, speech intelligibility, and music
clarity. There are a number of metrics that can provide this information. These
are interpretations of the measured data and generally correlate with subjective
perception of the sound at that seat.
8. An often overlooked method of evaluating the impulse response is the use
of convolution to encode it onto anechoic program material. An excellent freeware
convolver called GratisVolver is available from www.catt.se. Listening to the
IR can often reveal subtleties missed by the various metrics, as well as provide
clues as to what postprocess must be used to observe the event of interest.
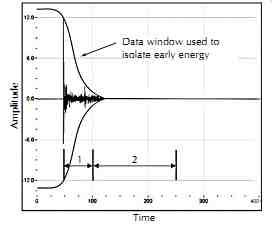 FIG. 23. A data window is used to remove the effects of the later arrivals.
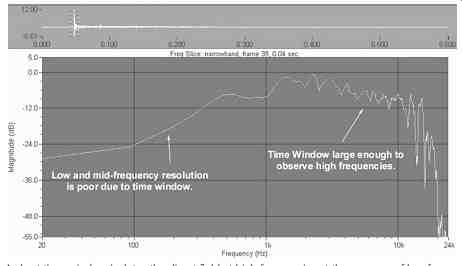 FIG. 24. A short time window isolates the direct field at high frequencies
at the expense of low-frequency resolution.
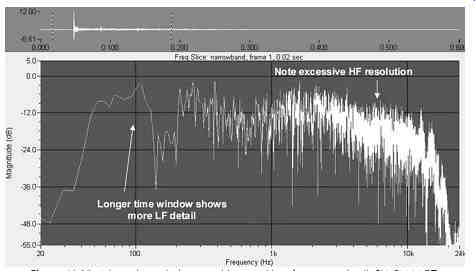
FIG. 25. A long time window provides good low-frequency detail.
3.6 Human Perception
Useful measurement systems can measure the impulse response of a loudspeaker/room
combination with great detail. Information regarding speech intelligibility
and music clarity can be derived from the impulse response.
In nearly all cases, this involves postprocessing the impulse response using
one of several clarity measure metrics.
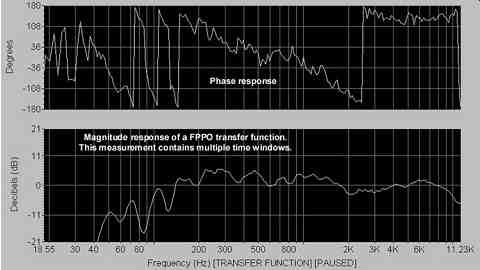 FIG. 26. The time window increases with length as frequency decreases.
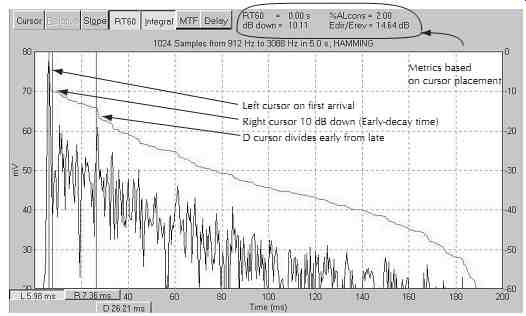 FIG. 27. The ETC can be processed to yield an intelligibility score, TEF25.
3.6.1 Percentage Articulation Loss of Consonants- (%Alcons)
For speech, one such metric is the percentage articulation loss of consonants,
or %Alcons. Though not in widespread use today, a look at it can provide insight
into the requirements for good speech intelligibility. A %Alcons measurement
begins with an impulse response, which is usually displayed as a log-squared
response or ETC. Since the calculation essentially examines the ratio between
early energy, late energy, and noise, the measurer must place cursors on the
display to define these parameters. These cursors may be placed automatically
by the measurement program. The result is weighted with regard to decay time,
so this too must be defined by the measurer. Analyzers such as the TEF25 and
EASERA include best guess default placements based on the research of Peutz,
Davis, and others, FIG. 27.
These placements were determined by correlating measured data with live listener
scores in various acoustic environments, and represent a defined and orderly
approach to achieving meaningful results that correlate with the perception
of live listeners. The measurer is free to choose alternate cursor placements,
but great care must be taken to be consistent. Also, alternate cursor placements
make it difficult if not impossible to compare your results with those obtained
by other measurers. In the default %Alcons placement, the early energy (direct
sound field) includes the first major sound arrival and any energy arrivals
within the next 7-10 ms. This forms a tight time span for the direct sound.
Energy beyond this span is considered late energy and an impairment to communication.
As one might guess, a later cursor placement yields better intelligibility
scores, since more of the room response is being considered beneficial to intelligibility.
As such, the default placement yields a worst-case scenario. The default placement
considers the effects of the early-decay time (EDT) rather than the classical
T30 since short EDTs can yield good intelligibility, even in rooms with a long
T30. Again, the measurer is free to select an alternative cursor placement
for determining the decay time used in the calculation, with the same caveats
as placing the early-to-late dividing cursor. The
%Alcons score is displayed instantly upon cursor placement and updates as
the cursors are moved.
3.6.2 Speech Transmission Index-(STI) The STI can be calculated from the
measured impulse response with a routine outlined by Schroeder and detailed
by Becker in the reference. The STI is probably the most widely used contemporary
measure of intelligibility. It is supported by virtually all measurement plat
forms, and some handheld analyzers are available for quick checks. In short,
it is a number ranging from 0 to 1, with fair intelligibility centered at 0.5
on the scale.
For more details on the Speech Transmission Index, see the section on speech
intelligibility in this text.
3.7 Polarity
Good sound system installation practice dictates maintaining proper signal
polarity from system input to system output. An audio signal waveform always
swings above and below some reference point. In acoustics, this reference point
is the ambient atmospheric pressure. In an electronic device, the reference
is the 0 VA reference of the power supply (often called signal ground) in push-pull
circuits or a fixed dc offset in class A circuits.
Let's look at the acoustic situation first. An increase in the air pressure
caused by a sound wave will produce an inward deflection of the diaphragm of
a pressure micro phone (the most common type) regardless of the micro phone's
orientation toward the source. This inward deflection should cause a positive-going
voltage swing at the output of the microphone on pin 2 relative to pin 3, as
well as at the output of each piece of equipment that the signal passes through.
Ultimately the electrical signal will be applied to a loudspeaker, which should
deflect outward (toward an axial listener) on the positive-going signal, producing
an increase in the ambient atmospheric pressure. Think of the microphone diaphragm
and loudspeaker diaphragm moving in tandem and you will have the picture. Since
most sound reinforcement equipment uses bipolar power supplies (allowing the
audio signal to swing positive and negative about a zero reference point),
it is possible for signals to become inverted in polarity (flipped over). This
causes a device to output a negative-going voltage when it is fed a positive-going
voltage. If the loudspeaker is reverse-polarity from the microphone, an increase
in sound pressure at the microphone (compression) will cause a decrease in
pressure in front of the loudspeaker (rarefaction). Under some conditions,
this can be extremely audible and destructive to sound quality. In other scenarios
it can be irrelevant, but it is always good to check.
System installers should always check for proper polarity when installing
the sound system. There are a number of methods, some simple and some complex.
Let's deal with them in order of complexity, starting with the simplest and
least-costly method.
3.7.1 The Battery Test
Low-frequency loudspeakers can be tested using a standard 9V battery. The
battery has a positive and negative terminal, and the spacing between the terminals
is just about right to fit across the terminals of most woofers.
The loudspeaker cone will move outward when the battery is placed across the
loudspeaker terminals with the battery positive connected to the loudspeaker
positive.
While this is one of the most accurate methods for testing polarity, it doesn't
work for most electronic devices or high-frequency drivers. Even so, it's probably
the least-costly and most accurate way to test a woofer.
3.7.2 Polarity Testers
There are a number of commercially available polarity test sets in the audio
marketplace. The set includes a sending device that outputs a test pulse, FIG.
28, through a small loudspeaker (for testing microphones) or an XLR connector
(for testing electronic devices) and a receiving device that collects the signal
via an internal microphone (loudspeaker testing) or XLR input jack. A green
light indicates correct polarity and a red light indicates reverse polarity.
The receive unit should be placed at the system output (in front of the loudspeaker)
while the send unit is systematically moved from device to device toward the
system input. A polarity reversal will manifest itself by a red light on the
receive unit.
3.7.3 Impulse Response Tests
The impulse response is perhaps the most fundamental
of audio and acoustic measurements. The polarity of a loudspeaker or electronic
device can be determined from observing its impulse response, FIGs. 29 and
46-30. This is one of the few ways to test flown loud speakers from a remote
position. It is best to test the polarity of components of multiway loudspeakers
individually, since all of the individual components may not be polarized
the same. Filters in the signal path (i.e., active crossover network) make
the results more difficult to interpret, so it may be necessary to carefully
test a system component (i.e., woofer) full-range for definitive results. Be
sure to return the crossover to its proper setting before continuing.
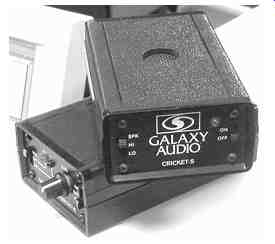 FIG. 28. A popular polarity test set.
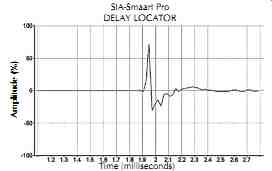 FIG. 29. The impulse response of a transducer with correct polarity.
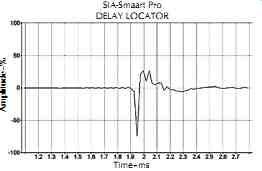
FIG. 30. The impulse response of a reverse-polarity transducer.
4 Conclusion
The test and measurement of the sound reinforcement system are a vital part
of the installation and diagnostic processes. The FFT and the analyzers that
use it have revolutionized the measurement process, allowing sound practitioners
to pick apart the system response and look at the response of the loudspeaker,
roo, or both. Powerful analyzers that were once beyond the reach of most technicians
are readily available and affordable, and cost can no longer be used as an
excuse for not measuring the system. The greatest investment by far is the
time required to grasp the fundamentals of acoustics to allow interpretation
of the data. Some of this information is general, and some of it is specific
to certain measurement systems.
The acquisition of a measurement system is the first step in ascending the
capability and credibility ladder.
The next steps include acquiring proper instruction on its use by self-study
or short course. The final and most important steps are the countless hours
in the field required to correlate measured data with the hearing process.
As proficiency in this area increases, the speed of execution, validity, and
relevance of the measurements will increase also. While we can all learn how
to make the measurements in a relatively short time span, the rest of our careers
will be spent learning how to interpret what we are measuring.
|