THE IMPORTANCE OF THE POWER SUPPLY UNIT
In the early days of audio, when mains-powered amplifiers were based almost
exclusively on the use of thermionic valves, very great attention was paid
to the design of the power supply unit from which the amplifier was fed. This
was mainly in order to reduce the extent of the 100 or 120 Hz mains 'hum'
whose presence, to some degree, was an almost inevitable accompaniment to
the audio output of the system.
In more recent times, when low-cost audio systems have been exclusively based
on 'solid-state' devices (bipolar junction transistors or ICs) the relatively
high degree of power supply line signal rejection -- referred to as PSRR (power
supply rejection ratio) in the IC manufacturers' data sheets - normally found
in solid-state circuitry has encouraged a much more off-hand approach to this
aspect of system design. This is a mistake, in design terms, since the output
signal, usually developed in respect of an 'earthy' 0-volt line, is applied
to the load in series with the power supply unit. This is true, as shown in
FIG. 1, whether the output is single-ended (as in FIG. 1(a)), or 'direct coupled,
and balanced about the 0V rail (as shown in FIG. 1(b)). Failure to consider
the way in which the output current flow path was completed led to a design
oversight in a commercial 'pseudo-class A' amplifier which was analyzed in
an article in Electronics + Wireless World (JLH, EWW, December 1989, pp. 1167-1168).
In the example considered, the power amplifier operated in class A at a high
quiescent current, but using a low voltage power supply to minimize the thermal
dissipation in the output transistors. The 0V line of the low-voltage power
supply unit which fed this amplifier was not connected to the main, LS return,
0V line, but was taken to the output of a higher power class B amplifier whose
function was to move the whole low-voltage supply, bodily, in relation to
the 0V line. The intention of this arrangement was that the class A amplifier
would be able to provide a high LS output voltage swing without a high dissipation.
However, this meant that the LS return path to the 0V line was now taken via
the class B amplifier as well, and any distortion in this would appear in
the output to the LS.
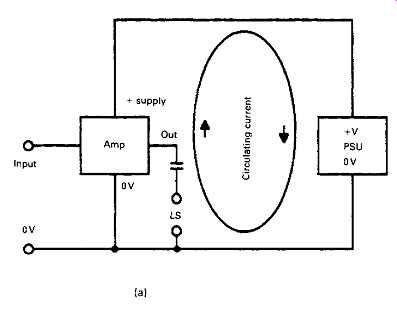
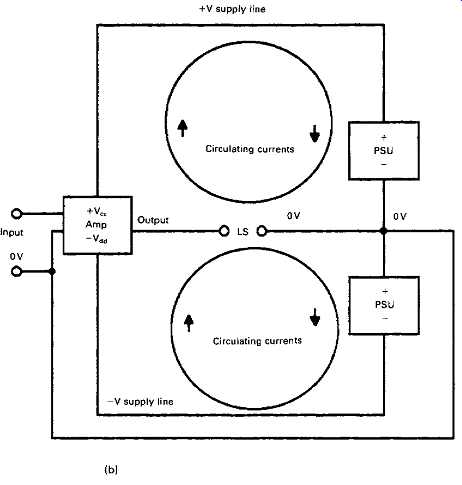
FIG. 1 Amp/PSU current flows, (a) Single-ended system; (b) direct-coupled
system.
CIRCUIT LAYOUTS
The simplest way of converting an input AC voltage waveform of the kind shown
in FIG. 2(a) into a DC voltage output is to pass it through some device which
will only allow current to flow in one direction (normally called a 'rectifier')
as shown in FIG. 2(c). By the choice of a suitable power transformer and rectifier,
any required output current or voltage could be obtained. Unfortunately, as
illustrated in FIG. 2(b), the output would be a series of uni-directional
current pulses. This is satisfactory for operating lamps or motors, perhaps,
but not for electronic equipment or audio systems.
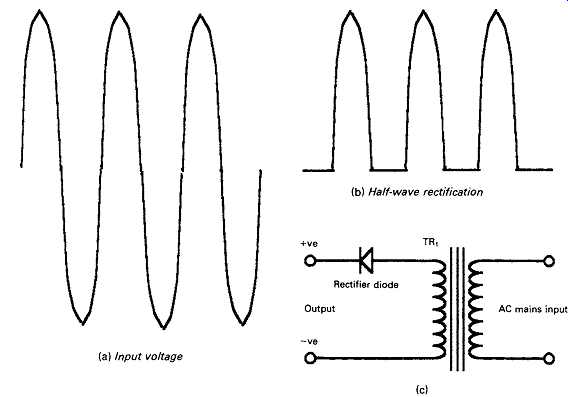
FIG. 2 Simple half-wave rectifier system. (a) Input voltage --- (b) Half-wave
rectification
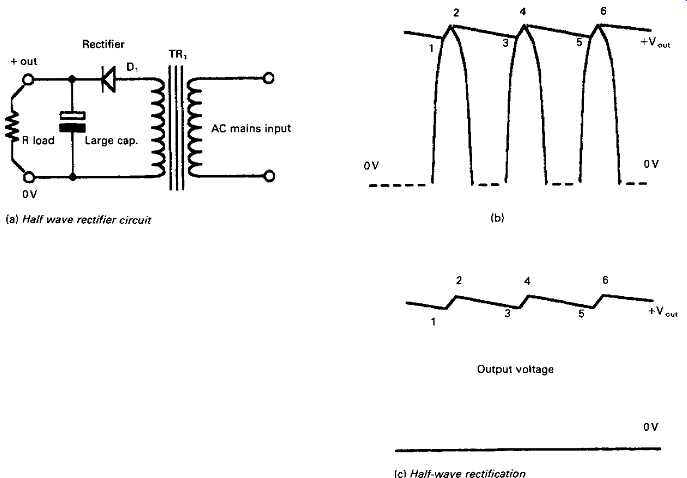
FIG. 3 Half-wave rectifier output ripple. (c) Half-wave rectification (a)
Half wave rectifier circuit (c) Half-wave rectification -- Output voltage
This situation is improved if an output 'reservoir' capacitor is included
in the circuit, as shown in FIG. 3(a), to store the output voltage until the
following capacitor charging pulse. However, this is still not a complete
answer to the design requirement for a smooth, hum-free output voltage, since
the voltage stored in this capacitor will decay as current flows through the
external load resistor, as shown in FIG. 3(b). The transformer/ rectifier
circuit can only recharge this capacitor when the peak transformer output
voltage somewhat exceeds the voltage on the capacitor, as is the case between
the points labeled 1-2, 3-4, 5-6 and so on. This leaves an undesired ripple
on the output DC voltage waveform shown in FIG. 3(c), which worsens , for
a given size of reservoir capacitor, as the output current demand is increased.
Unfortunately, this recharging current flows only for a brief period each
cycle, and it must be larger than the mean output current by the ratio (cycle
duration):(conduction duration). If the reservoir capacitor is large, the
rectifier diode has a low conducting resistance, and the transformer is of
high quality with a low resistance windings then the peak charging currents
when the diode is conducting, between points 1-2, 3-4, etc., will be very
high.
CIRCUIT PROBLEMS
No electrical circuit has zero resistance (R) at room temperature, and the
voltage (V) developed for a given current flow (I) will be V = IR. In a typical
power supply the reservoir capacitor charging currents could well be hundreds
of amperes, and this will cause a significant voltage drop, in addition to
that due to the circuit resistances, due to the equivalent series resistance
(ESR) in the reservoir capacitor. The voltages produced by these pulsating
currents will be added to the existing ripple voltage present on the power
supply output line.
A further problem of which the power supply unit (PSU) designer must take
note is that the very high short-duration circulating currents, during the
recharging of the reservoir capacitor, can generate troublesome voltages across
all parts of the wiring (or chassis connections) which are in the path from
the transformer to the rectifier, to the reservoir capacitor and back to the
transformer again. If any part of the signal chain shares any part of this
path, it will have some of this 'spiky' short-duration hum voltage added to
it. (A further nuisance is that these high peak currents cause strong, short-duration,
magnetic fields, which can induce undesired voltages in adjacent wiring.)
Unfortunately, all the steps that the designer can take to lessen the ripple
on the PSU output, of which the most common is the choice of a very large
value, low ESR reservoir capacitor, will tend to make the charging duration
shorter and thereby increase the pulsating peak current flow. High pulsating
currents also make demands on the construction of the mains transformer -
an aspect which I will consider later.
FULL-WAVE RECTIFIER SYSTEMS
An immediate improvement in the performance of any simple transformer/ rectifier
system can be made if both halves of the AC output waveform are used, rather
than only, say, the positive-going half. This technique, in its various forms,
is almost universally employed in PSU systems since it halves the duration
of the conducting cycle, and thereby also halves the ripple voltage and the
peak reservoir (and transformer secondary) pulse currents.
With valve rectifiers, where it’s necessary to isolate the cathode/heater
circuits, the circuit normally used was of the kind shown in FIG. 4(a) for
an indirectly heated rectifier valve such as a 5Z4. This kind of valve is
to be preferred to a directly heated rectifier, such as the 5U4, if an adequate
output current could be obtained, because the HT voltage would only rise gradually
following switch-on, as the cathode gradually warmed up to its operating temperature.
However, indirectly heated cathodes are less efficient for a given heater
wattage, and will have a somewhat shorter operating life.
A fairly common solid-state equivalent system is shown in the circuit of
FIG. 4(b). The transformer secondary voltage and ripple waveforms for these
types of circuit are shown in Figs 4(c) and 4(d). With solid-state rectifier
systems, in which the designer is free from the constraints imposed by the
need for heater connections and supplies, other layouts are possible, such
as the full-wave bridge rectifier layout shown in FIG. 5(a), used here to
provide a single-polarity output voltage. This has an advantage over the layout
shown in FIG. 4(b) in that instead of each half of the secondary winding conducting
alternately, both secondary windings conduct each half-cycle. This reduces
the ratio of peak-to-mean current flow in the transformer secondary circuit,
and helps to lessen both the audible hum and the electro-magnetic field radiation
from the transformer. However, while this reduces the voltage drop in the
secondary windings due to the high peak-current flow, it requires the presence
of two rectifier diodes in the path from secondary winding to DC output, instead
of just one.
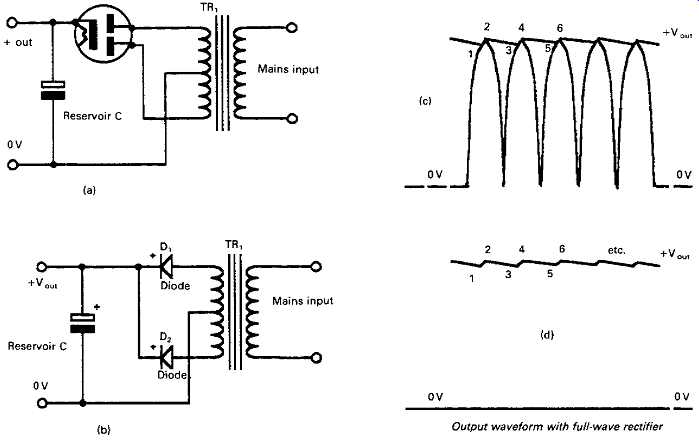
FIG. 4 Full-wave rectifier systems. Output waveform with full-wave rectifier
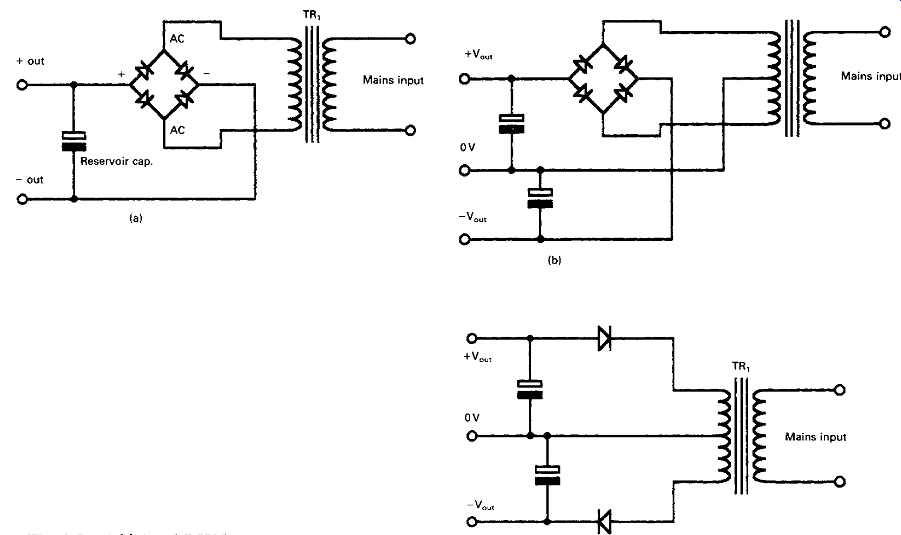
FIG. 5 Additional PSU layouts.
For a twin-polarity power supply output, the most usual circuit layout is
that shown in FIG. 5(b), which shares the advantages of more complete utilization
of the current output from the secondary winding and also that of lower peak
current levels. Both these layouts will give the type of ripple voltage shown
in FIG. 4(d). The half-wave rectifier circuit shown in FIG. 5(c) is sometimes
found in low-current twin-rail supply systems, where the major considerations
are those of simplicity and cheapness. It has the output ripple characteristics
shown in FIG. 3(c). The choice of the size of the reservoir capacitor in any
of the preceding circuits demands some compromise. The desire to keep the
output ripple voltage to the lowest possible level - coupled with the wish,
particularly in the case of audio amplifiers, to arrange the PSU so that it
can deliver high peak output currents, where required - urges the choice of
as large a value of reservoir capacitor as the space and money available will
allow.
However, increasing the size of the reservoir capacitor causes the current
from the transformer secondary to be drawn in increasingly short pulses, which
will worsen the transformer efficiency and heat dissipation. In practice ,
for any given transformer, the inevitable secondary winding resistance and
leakage inductance, associated with core saturation effects, means that there
is an optimum reservoir capacitor size, beyond which it’s not useful to go.
TRANSFORMER TYPES AND POWER RATINGS
A major pitfall which lies in wait for the unwary PSU designer is that relating
to the maximum output current which may be drawn, without overload, from any
circuit with an output reservoir capacitor - such as , for example, those
illustrated in Figs 3(a) or 4. The DC output from these layouts will approach
that of the peak transformer secondary voltage (approximately 1.4 times the
secondary RMS voltage figure, less any voltage drop due to the rectifier or
the secondary winding resistance, and less the mean output capacitor voltage
droop between recharging cycles). The transformer secondary winding will then
be required to deliver a peak current to the reservoir capacitor which is
equal, when averaged, to the mean DC drawn from the system. The transformer
is then delivering an output power which is equivalent to V peak x 7out rather
than VRMS X ???? > and this may be in excess of the manufacturer's rating.
A working 'rule of thumb' is that the DC output current (/DC) should not exceed
the specified /RMS (max.) x 0.65.
It’s desirable in any mains input transformer that there should be an intimate
magnetic coupling between all the windings, and that there should be no stray
magnetic field around it. The extent to which this ideal is achieved depends
greatly on the nature of the core material (usually grain-oriented silicon
steel), and the type of core construction.
In low-cost transformers this core is made by assembling, with the least
practicable air gaps in the magnetic circuit, a suitable sized stack of thin,
flat, steel alloy 'laminations' - which have been stamped out in the form
of 'E's and Ts - within an appropriately shaped hole in an insulating former
holding the windings. The laminations must be tightly clamped to prevent core
'buzz', after which the whole assembly will be dipped in some moisture-proofing
and insulating lacquer. In higher-quality contemporary transformers the core
will be an annular, 0-shaped 'toroid', wound from a continuous thin strip
of high-grade magnetic material. The windings are wound around this core by
a specially designed machine prior to dipping and impregnation.
Because of the superior magnetic circuit characteristics of the toroidal
core, there is much less magnetic field radiation from such a transformer,
and it has a superior electrical performance. In high-quality audio equipment
these factors will greatly outweigh its less compact construction and its
greater cost. Also, because of the way the transformer and its core is made,
there is much less core or winding 'buzz' in use - a factor which is welcomed
by those with keen ears.
STABILISED PSU CIRCUITS
Low power
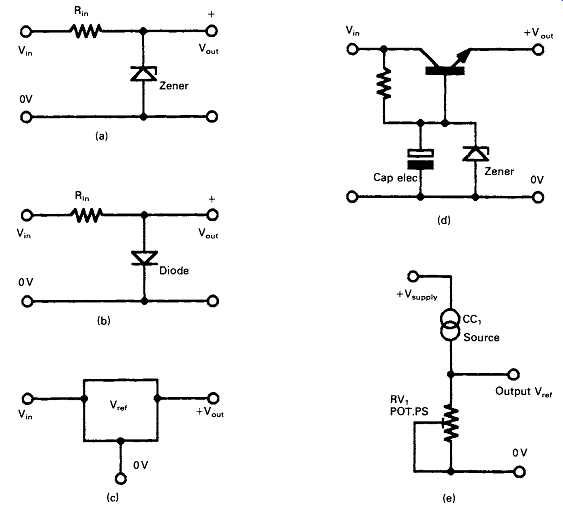
FIG. 6 Voltage reference sources.
There are a number of places in audio amplifier circuitry where a source
of some fixed, noise and ripple-free voltage is needed. Some of the circuit
layouts used for this purpose are shown in FIG. 6. The most common general-purpose
circuit is that using a 'zener' diode, as shown in FIG. 6(a). (The term 'zener'
is used loosely to describe all devices of this type, though, strictly, zener
diodes are those with output voltages in the range of about 3-5.5 V. Above
this voltage they are more properly described as 'avalanche diodes'.) All
these reverse-breakdown devices generate a significant amount of electrical
noise, and it’s usually prudent to connect a capacitor in parallel with the
zener to reduce the noise output as , for example, in FIG. 6(d). If the circuit
requires only a relatively low voltage output, a much better circuit, from
the point of view of noise, is that of FIG. 6(b), using one or more silicon
junction diodes connected in series in the forward (conduction) mode. Multiples
of the forward voltage (usually around 0.57-0.6 V) can be obtained in this
way. For high-precision, low temperature coefficient applications there is
a wide range of voltage 'bandgap' reference sources, such as the ICL8069 or
the LM369, which offer very high precision, low-noise, reference sources in
the range 1.22-10 V, but these would be rather too expensive for general audio
use.
A very simple means of providing a small adjustable reference voltage --
commonly used to generate the forward bias on the output devices in a transistor
amplifier - is that shown in FIG. 6(e). The thermal characteristics of the
constant current source, CQ, should be chosen to suit the particular application.
Higher power:
Although some manufacturers still prefer to use 'in-house' designs for voltage
regulators based on discrete components, the 'three-terminal' voltage regulator
ICs of the '78../79..' or '78L../79L..' types have now become an almost inevitable
choice for use in relatively low voltage (up to, say, ±24 V) supplies for
use with low-level signal circuitry, in preamps or tape/CD/radio systems.
The advantages of these ICs are significant. They are cheap, compact, predictable,
compensated for variations in ambient temperature, available in both +ve and
-ve line versions and have in-built overload protection. They also have a
very high degree of power supply ripple rejection, and a low output noise
- especially the low-power 'L' series. Although higher-power and higher-voltage
versions are becoming increasingly available, they are not, as yet, readily
available for use at the voltage, current and power levels needed for audio
power amp supplies.
Therefore, in this field, if voltage regulation is required, it will be in
the form of a discrete component circuit.
However, the question of whether to use a voltage regulator system opens
up philosophical divisions within the audio amp manufacturers, based on their
judgment of their likely market. If the power output stage of an audio amplifier
is fed directly from a large reservoir capacitor and a massive transformer/rectifier
combination, of the general type shown in FIG. 5(b), it will be able to deliver
very high powers into the LS load, especially under short-duration signal
demands - the origin of the so-called 'music power' ratings of the 1960s.
This capability is particularly welcomed by those for whom the physical impact
of high-level sound is almost as important as the artistic content of the
signal. For those users any regulator circuit which acted to limit the off-load
power supply voltage, and the consequent peak sound level output, would be
very unwelcome.
Unfortunately, there are also snags. If the power supply can deliver large
output currents at high voltages into the LS load, under short-duration music
conditions, it will also be able to do it for a longer time under fault conditions,
which could be destructive of expensive LS reproducers. The normal type of
damage limitation offered by the manufacturers of amps using unprotected PSUs
is to incorporate a suitable 'slow-blow' fuse in the output circuit, coupled
possibly with some fast-acting overload 'crowbar' circuit to short-circuit
the amp output under overvoltage fault conditions.
The snag with the use of any output line fuse is that the integrity of the
fuse/fuse-holder contacts will deteriorate with time, and will then introduce
unwanted resistance into the LS output circuit.
Some rather less obvious design problems are that the residual (100/ 120
Hz) AC ripple on the PSU output will increase as the output current rises,
and this will increase the amplifier background hum level at high output powers.
This may not be very noticeable because it will be masked by the music output,
but it will be measurable under tests using spectrum analysis, and may be
commented upon by suitably equipped reviewers.
An additional problem, more apparent to the designers than to the users,
is that the off-load output voltage of such a simple supply system can be
up to 40% greater than that on load. This means that components in the power
amp will need to support the full off-load voltage, plus some safety margin
to allow for mains input voltage surges rather than the supply voltage existing
under full-load conditions. These components could be more expensive and,
in the particular case of transistors, will usually be of a rather lower electrical
performance.
For an audio system which is likely to be used mostly with more traditional
music, of which the aesthetic appeal may rely less upon physical impact than
upon clarity and tonal purity, a system in which the power amplifier is fed
with constant voltage smooth DC supplies, and in which the output power is
the same for sustained signal outputs as it’s for brief-duration transients,
has much to commend it. However, it may be less well liked by the 'heavy metal'
or 'reggae' devotees.
Higher power regulator layouts:
These can be divided into 'shunt' and 'series' connected circuits, of the
kinds shown in Figs 6(a) and 6(b) respectively, of which the simple zener
diode circuit of Fig. 6(a) is the best-known type. Although zener diodes
are available with a range of permissible dissipations from 400 mW to some
tens of watts, the higher-power versions are not widely available and expensive.
The circuit shown in FIG. 7(a) uses a combination of a power transistor, Q1,
with a low-power zener diode to give a high-power shunt regulator circuit.
The operating voltage of the zener diode needs to be chosen so that the circuit
will draw current from the power supply and reduce its output voltage (due
to the voltage drop across its source resistance, Rin), at some point between
its off-load and full power voltage levels.
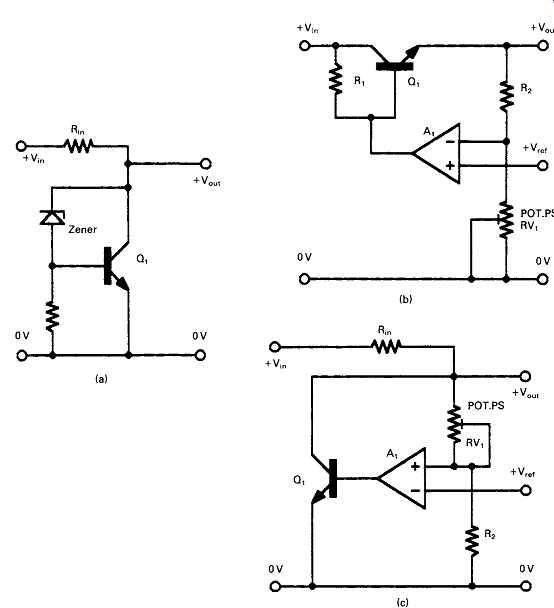
FIG. 7 Shunt and series regulators.
A more elegant circuit arrangement is shown schematically in FIG. 7(c), where
the difference between some stable reference voltage and a proportion of the
output voltage is amplified and fed to a power transistor connected across
the power supply output. The precise operating voltage for this circuit can
be chosen by setting the preset potentiometer RVx.
A typical series voltage regulator circuit is shown in FIG. 7(b), in which,
once again, a proportion of the PSU output voltage is compared with a reference
voltage, and an amplifier, A1, is used to adjust the current fed into the
base of the series 'pass' transistor, Ql5 so that the required output voltage
is obtained. The snag with a series voltage regulator of this type is that
, for correct operation, the input voltage must exceed the required output
voltage by some 3 V - known as the 'dropout' voltage - or otherwise the current
flow through R1 won’t be adequate to support the required output current.
In the variations of FIG. 7(b) which are available in IC form, the voltage
reference is invariably derived from a 'band-gap' type of temperature-compensated
voltage source. Not only is the output voltage derived from this arrangement
much more stable than that from a zener diode, it’s also much more noise free.
This is a point which is sometimes overlooked by designers who use a 'quiet'
IC regulator to provide a lower voltage to drive a 'noisy' zener diode.
Discrete component voltage regulators can be built to provide any required
combination of output voltages and currents, and I am convinced that their
use to drive audio amplifier systems does provide worthwhile benefits in sound
quality, if for no other reason that such a circuit can have an output impedance
which is largely frequency independent and can be less than a tenth of one
ohm. To obtain such an output impedance, at, say, 20 Hz from any output capacitor
would demand a capacitance value of some 80000 µ¥ - a very bulky and costly
alternative. A further advantage of such an electronically controlled supply
system is that it can be made to current limit to protect the circuit components
from overload, or cut off the supply completely in the event of any amplifier
failure. For my own use, I greatly prefer such systems, and described two
such layouts for use with power amplifier designs in Electronics + Wireless
World (May 1989, pp. 524-527) and Electronics Today (May 1989, pp. 25-33),
shown respectively in Figs 8 and 9.
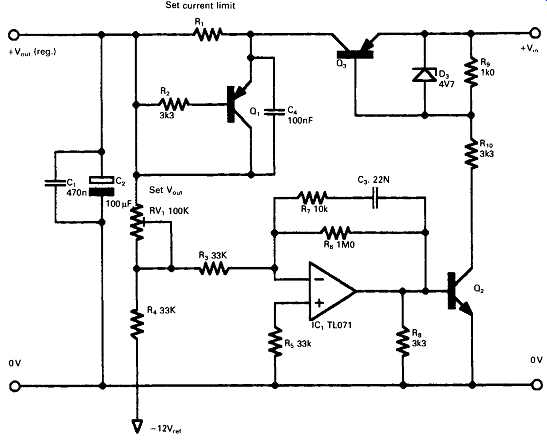
FIG. 8 Series-stabilized PSU.
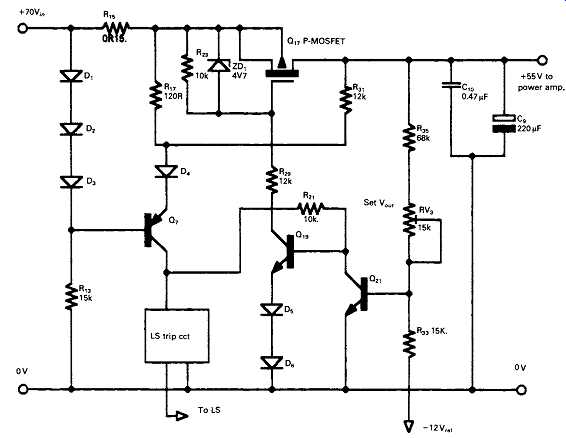
FIG. 9 SIC protected stabilized PSU.
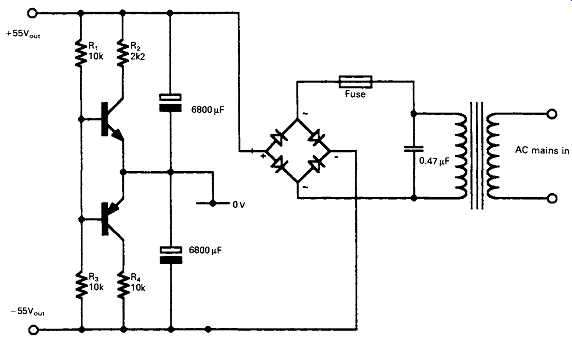
FIG. 10 The Quad power supply system.
COMMERCIAL POWER AMP PSUs
At one time, most of the more highly esteemed audio power amplifiers would
have employed some form of PSU output voltage regulation.
However, this approach has now been largely abandoned, I suspect as a result
of market pressures, in favor of simpler layouts whose principal feature is
an ability to provide the high peak supply line currents needed for some modern
music. The PSU circuit details given in the data sheets for the bulk of contemporary
power amps show only variations of the simple layout of FIG. 5(b), coupled
with some overcurrent protection, or relay switching, circuit to give a measure
of LS protection.
Nevertheless, some interesting variations still exist, such as the use by
'Pioneer' of a version of the augmented zener diode layout of FIG. 6(d) as
the main power supply line stabilization circuit for their M90(BK) flagship
amplifier, an arrangement which is also used by Technics and Rotel. An ingenious
system shown in FIG. 10 is used by 'Quad' (the Acoustical Manufacturing Co.)
in many of their current power amp designs for converting a single polarity
supply into a pair of symmetrical ± voltage lines.
OUTPUT SOURCE IMPEDANCE AND NOISE
Bearing in mind that the output of any amplifier will drive its load in series
with its power supply source resistance, and that a proportion of the noise
present on the DC power supply lines may also appear on the output signal,
the output impedance and noise characteristics of power supply units are of
considerable interest to the circuit designer. The commercial three-terminal
voltage regulator ICs have a lower noise level and output resistance than
small signal zener diodes. They are preferable where these characteristics
are important, as , for example, in low-level audio circuitry.
Both primary and secondary cells have good electrical characteristics when
new, and the performances of these are listed in Table 9.1.
Table 9.1 Cell and voltage regulator characteristics Notes. The quoted noise
levels were measured over a 20 Hz to 20 kHz bandwidth at 20°C. All the (AA
size) battery cells had a noise voltage which was below 1 µV on a 10 mA current
drain. The '79' series regulators have a somewhat inferior performance by
comparison with the '78xx' types. This is because it’s difficult to make good
p-n-p transistors in IC form, and this necessitates the use in the *79xx'
devices of a somewhat different internal circuit layout.
TRANSFORMER NOISE AND STRAY MAGNETIC FIELDS
All mains transformers generate some audible noise as a result of the alternating
magnetic flux and the consequent mutual magnetic repulsion effects within
their core and windings, and this can, sometimes, be annoying. E and I type
lamination assemblies, used to construct the magnetic cores in conventional
low-cost mains transformers, are less good in this respect than the toroidal
and 'C-core' constructions used in high-quality equipment. The use of a higher
quality core system leads to a much lower external (stray) magnetic field,
which facilitates the design of mains-operated units having a low background
'hum' level, and also makes units employing toroidal or similar core transformers
less likely to induce mains 'hum' into the circuitry of other units placed
in proximity to them.
However, the ability of the core of a transformer to confine the associated
magnetic field also depends on the permeability of the core material and this,
in turn, depends on the magnitude of the flux within the core. If this approaches
saturation the permeability will fall, and the external magnetic field will
increase. For this reason the stray magnetic field in proximity to the transformer,
as well as its noise level, will increase as the peak currents in its windings
increase, and this is a drawback in the use of very large value power supply
reservoir capacitors unless this is accompanied by an increase in the power
rating of the component. |